Death is a complex affair, at least for cells. There are several ways in which a cell can die: It might commit a form of suicide known as apoptosis, for example, or self-digest by necrosis. Further complicating matters is the fact that some cells may appear dead as doorknobs although they’re actually in a limbo between life and death—a state from which they might at some point return as transformed versions of their old selves.
An intriguing example of such cellular zombies was recently discovered in the lab of Paul Greengard, a Nobel laureate and Rockefeller’s Vincent Astor Professor, who passed away last year. A team of scientists was trying to figure out what goes wrong in the brains of people with Parkinson’s disease. The researchers had long struggled to understand why dopamine-producing neurons in the midbrain perish, leading to debilitating movement problems characteristic of Parkinson’s.
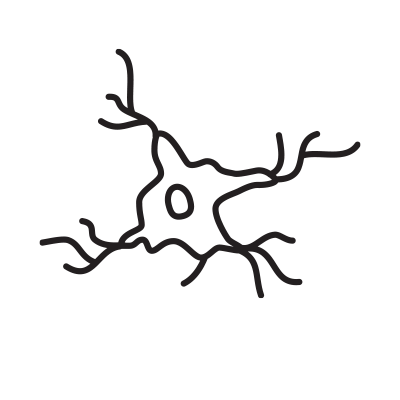
Data
Neurodegeneration happens in all people, all the time. On average, an adult loses 3,250 neurons every hour.
In retrospect, they may have been asking the wrong question. As the scientists reported in Cell Stem Cell, at least some of these midbrain neurons appear not to be dead after all, but rather to be resting in a zombie-like state known as senescence. And the results suggest that a zombie neuron may be even more damaging to the central nervous system than a dead one: By releasing inflammatory chemicals, the undead cells spread senescence to surrounding healthy neurons and make those neighbors shut down as well.
Research associate Markus Riessland says the discovery was especially surprising given that senescence is almost unheard of among neurons, although it does occur frequently in other parts of the body.
“Our findings shed new light on how Parkinson’s disease progresses,” he says, “and might provide new opportunities for treatment.” For example, Riessland and his colleagues suspect that so-called senolytic drugs, which are known to remove senescent cells, might make it possible to slow the brain’s deterioration.