Feature
Frames of Mind
If the key to a life well lived is good decisions, the key to good decisions is flexibility. Behind the brain’s remarkable adaptability is its ability to reach vastly different conclusions based on the same information. Neuroscience, we are learning, is even more complex than we thought.
By Bahar Gholipour & Alexander GelfandIf there’s one thing we know about insects, it’s that they’re drawn to light. It’s why streetlights attract clouds of gnats, ceiling fixtures become insect graveyards, and bug zappers emit that purple glow. Take one dark summer night, add a little candlepower, and the nearest 200 insects will always appear.
Except, it turns out, they don’t always appear. If you conduct a controlled experiment with a single fruit fly and a single light source, you’ll discover that the fly sometimes flies toward the light and sometimes doesn’t. It might instead wander aimlessly, find a dark space to rest, or not react at all. Although light attracts insects, it doesn’t do so consistently.
This is a problem for neuroscientists.
The brain, we’ve been taught, is supposed to work like a web of circuits. A sight, sound, or smell goes in, connections are activated between neurons, and a behavioral response emerges. But why doesn’t the same stimulus result in the same response every time? Even in the simplest organisms, neurologically speaking, those with only a few hundred neurons in their bodies, the exact same stimulus in the exact same situation can result in a range of behaviors.
In other words, the brain has a talent that scientists have yet to wrap their heads around. It seems capable of toggling its own circuitry when the need arises. It makes sense, of course—the brain’s ability to produce robust behaviors is critical for survival, but these behaviors also need to be flexible.
Otherwise we’d all be robots stuck in a loop.
For us humans, there are words like “mood” and “feelings” to describe the brain’s flexibility, depending on, for instance, how tired, hungry, lonely, stressed, or anxious we are. It’s anyone’s best guess whether flies feel changes in their internal weather the way we do, but all organisms have motivations. They may or may not suffer from hunger, for example, but they certainly have a stronger drive to find food when it’s been a long time since their last meal.
Some scientists refer to these neurological milieus as “internal states,” relatively long-lasting shifts in the brain’s inner activity that temporarily change how one interacts with the world. The existence of internal states isn’t news per se. A century ago, ethologists who famously described how certain behaviors are innate, hardwired into the nervous system prior to any learning, also noticed that these behaviors are too complex to be explained as chain reflexes. They observed in animals such as fish, birds, and bees innate patterns of behavior, such as foraging and fighting, that were triggered by specific sensory stimuli—the smell of a potential mate or the sight of a potential rival. But depending on the circumstances, the same sensory cue could sometimes elicit one type of behavior, sometimes another, sometimes none at all. These early pioneers could only hypothesize about what happens in the brain to create such versitality—and in the decades that followed, biologists had neither the basic understanding nor the tools to penetrate this question. Even with recent connectomics studies in which the wiring diagrams of some organisms’ nervous systems were completely mapped out, scientists have been left with more data than answers.
Now, at long last, they may be ready to explore the brain in a new light.
Aided by new technology that makes it possible to peer deeper into the nervous system with more control and precision, a number of labs are venturing into the vast abyss separating our knowledge about the brain’s hardware and an individual’s fluid behavior. These researchers are now taking the first steps to outline the mechanisms by which internal states such as hunger and arousal influence the behavior of organisms as simple as worms and as complex as us, shaping the way we respond to our environments and to other creatures.
Even in the simplest organisms, the same stimulus in the same situation can result in a range of behaviors. the brain, it seems, is capable of toggling its own circuitry when the need arises.
“We still don’t know how the brain really works,” says neuroscientist Cori Bargmann, who studies decisionmaking in the Caenorhabditis elegans nematode. “We are able to describe how information flows through an individual synapse, and we know that some brain regions are important for specific behavioral functions. But what happens in between? How does information from thousands of firing neurons get organized, and how does this organization fluctuate over time?”
Looking at individual neural circuits is useful, Bargmann says, but unless we consider the larger context in which those circuits are operating we will see only a tiny piece of the picture.
“Internal states are an incredibly important feature of the brain that has been understudied,” she says. “And I believe it will have clinical relevance for mood disorders, drug addiction, and a host of other intractable brain diseases.”
In a small, darkened room deep inside Vanessa Ruta’s lab, tens of thousands of flies are ready to surprise us. Bred in vials about the size of a glue stick, these Drosophila melanogaster flies—prime subjects of genetic studies for over a century—have all kinds of interesting traits not found in the wild. Some are missing genes tied to odorant receptors, some lack the neurons they need to process visual information, and so on.
By testing various flies in different behavioral scenarios, Ruta and her team can piece together how specific types of neurons work together to influence behavior. And by using flies whose neurons are modified to express a fluorescent protein when they fire, she can literally watch what happens in a male fly’s brain when it encounters a female.
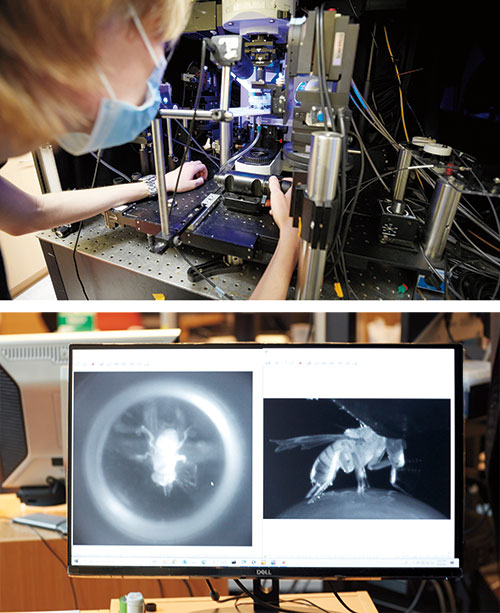
Flies are dogged suitors when they want to be: A male fruit fly will pursue a female for more than 26 yards—the equivalent, in human terms, of nine miles—if he’s eager to mate. If he’s not, he might not even notice her. Same individual, same conditions, same preprogrammed behavior—but a different response.
Ruta uses a fly-size virtual reality stage to watch this behavior unfold. Picture a male fruit fly tethered to a little foam ball covered in tiny black dots and floating atop a stream of air. A curved screen placed directly in front of the animal covers its entire field of view, and there is a camera behind the fly and a high-resolution microscope positioned directly above its head.
By projecting a dot onto the screen and mimicking the characteristic movements of a female fly, the researchers can trick a male into believing he has encountered a potential mate. An interested, aroused male will begin walking toward the fictive female, spinning the foam ball in the process. As a camera tracks its movement, the overhead microscope records the activity of his fluorescing neurons.
The neurons Ruta is interested in today, known as P1 neurons, are known to create the arousal that gets the fly interested and moving. Turn them on and the fly pursues a simple moving dot in front of him. Shut them down and he won’t pursue anything.
Ruta’s graduate student, Tom Hindmarsh Sten, found that when P1 neurons are turned on, visual neurons sensitive to moving targets radically increase their firing and communicate with the fly’s motor system, enabling him to follow them. The activity of P1 neurons and signaling of the visual neurons work hand in hand, the researchers found, fluctuating together up and down for many minutes. In a way, the P1 neurons function like a dimmable switch modulating the intensity of communication between the fly’s vision and motor system.
“Even though these visual tracking neurons are detecting visual information all the time, we think they aren’t able to transmit it to the motor system unless the males are in an appropriately aroused state, as decided by the P1 neurons,” says Ruta, who is the Gabrielle H. Reem and Herbert J. Kayden Associate Professor.
How do the P1 neurons determine whether it’s an appropriate time to be aroused? Ruta speculates that these neurons integrate signals from the flies’ sensory organs—visual, auditory, odorant, etc.—suggesting the presence of a viable mate, plus, perhaps, the fly’s own physiological state such as hunger or tiredness.
The design makes a lot of sense. This segregated circuit logic reconciles two competing needs of the fly: first, to enable the fly to reliably track and pursue a mate and, second, to remain sensitive to moment-to-moment feedback, allowing him to change his behavior if it’s no longer appropriate. The internal state that we call arousal is nature’s solution for producing behaviors that are at once robust and flexible.
Taken together, these findings present one of the clearest and most detailed pictures to date of how a motivational state is created, how it changes the way information is processed and routed in the brain, and how it ultimately influences behavior.
For some neuroscientists, flies are far too complicated, with their 100,000 neurons. A simpler model organism, they contend, is the best way to link individual neurons to individual behaviors. For these researchers, there is the 302-neuron C. elegans worm, in which every neuron has been mapped and every gene decoded.
With C. elegans, scientists such as Bargmann have the whole brain and the entire animal. They can see the whole system at once. And indeed, in many cases, the worm’s response to a stimulus is quite simple.
For instance, take their response to diacetyl. Worms are easy to attract with this common chemical—the same compound that gives popcorn its buttery smell. Bargmann knows how to manipulate the receptor molecule for this odor in a neuron. Using genetic tools, she can make the molecule turn on in a different neuron; when that happens, the worms that detect diacetyl slither away from the source rather than toward it. This simple experiment—moving receptors around in neurons—shows how genes can be linked to specific neurons, and how neurons are prewired to behavior.
But not all the worm’s responses are as uncomplicated.
C. elegans, like many other organisms, uses pheromones to guide its social and sexual pursuits. But pheromones, mysteriously, sometimes attract companions into social groups and sometimes repel them. It turns out that the sensory neuron that detects pheromones is the same, but three circuits are involved in the decision: Bargmann’s lab found that one promotes attraction, another triggers repulsion, and the third decides which of the first two to listen to. By pitting the first two circuits against each other, boosting or suppressing their signals, this third input provides richer behavioral choices than those generated by the sensory neuron alone.
Pheromone responses are regulated not just by external sensory cues but by internal biochemistry—signaling molecules that “lobby” the neurons for one decision or another. The presence or absence of specific signaling molecules, which reflects the internal state of the worm’s tiny nervous system, helps shape the worm’s ultimate behavior.
“We wouldn’t know how this circuit computes just by looking at its wiring diagram. Despite having the complete anatomical description and connectome of C. elegans, we can’t see it as a set of absolute instructions,” says Bargmann, who is the Torsten N. Wiesel Professor. “Instead, the anatomy represents a set of potential connections that are shaped by context to allow different paths of information flow.”
It’s not just a maze. It’s a maze in which the paths between entrance and exit are reconfiguring themselves as you try to trace them.
Appetite is perhaps the classic example of an internal state. Feeling hungry? That smell drifting over from the neighbor’s barbecue is generating saliva, causing rumbles, and perhaps fostering a sudden desire to make new friends. Feeling full? Get that cheesecake out of sight before I puke. Figuring out the internal state of humans is exponentially more difficult than that of worms and flies. One reason for this is the layered, hierarchical nature of internal states. Our internal states have their own internal states.
For example, hunger is controlled in the hypothalamus by, among other factors, the hormone leptin. But conscious decisions can override hunger. People may decide to eat less to lose weight, or to eat more to bulk up. Every organism keeps tabs on its nutritional state and calorie reserve, and although much of this regulation is driven by circuits in the brain stem and in particular the hypothalamus—parts of the brain that highly resemble one another in all vertebrates—mammals have other parts of the brain that are (we like to think) more evolved.
“What’s different about mammals—in particular, humans—is we have a big cortex on top of these basic structures that regulates all the basic drives,” says Jeffrey M. Friedman. “The cortex then makes all kinds of other judgments that might to some extent influence the function of that basic simpler circuitry.”
Studying that simpler circuitry of hunger regulation, Friedman identified leptin 25 years ago. It was a breakthrough: a hormone that regulates food consumption and body weight. Manipulating leptin levels was the magic bullet that was going to allow us humans to adjust our appetites. But—and this is a recurring theme in neuroscience—it turned out not to be so simple.
Mental disorders—anxiety and posttraumatic stress disorder, for instance—look to neuroscientists very much like internal states run amok.
Leptin is produced by fat cells and suppresses appetite. Some people who suffer from obesity are in fact leptin deficient; thanks to Friedman’s discovery, leptin replacement therapy can help them lose weight. (Friedman derived the name leptin from the Greek word leptos, or “thin.”) But leptin affects more than food intake. Animals that are deficient in leptin are also less aggressive, less active, and less likely to engage in sex. And leptin isn’t the only player. Like arousal, hunger appears to be modulated by a complex web of interconnected biochemical and neural pathways.
“The hormone orchestrates a profound set of behavioral responses—and probably emotional responses—that then influence your response to all kinds of other stimuli,” says Friedman, who is the Marilyn M. Simpson Professor.
Friedman and others have identified a population of neurons in the hypothalamus that inhibits food intake when suppressed by leptin. In Friedman’s experiments in leptin-deficient mice, these neurons become hyperactive, ramping up food consumption. But in the cerebral cortex, which carries out higher brain functions, Friedman and his colleagues have recently discovered that a population of neurons can override the hunger circuitry of the hypothalamus, causing the animals to overeat even when they aren’t hungry.
And the nucleus accumbens, a key reward center in the brain that is implicated in addiction disorders, likely also plays a role in feeding behaviors. Neurons in the nucleus accumbens are activated by natural rewards like food and water as well as by drugs of abuse such as cocaine and heroin. By imaging large numbers of neurons in the nuclei of mice that were in withdrawal from cocaine and morphine, Bowen Tan, a student in the Friedman laboratory, and Tobias Noebauer, a fellow in the lab of Alipasha Vaziri, observed clear differences in the animals’ neural responses to food. The work explains how drug withdrawal, an internal state, influences the pleasure derived from eating.
Like Ruta’s findings regarding arousal in fruit flies, Friedman’s results provide an elegant illustration of how an internal state created by one neural circuit can be modified or inhibited by signals from another, adding flexibility to innate behaviors.
And because those behaviors are directly related to consumption, it’s still quite possible that manipulating these signals could lead to treatments for eating disorders and related conditions such as obesity and type 2 diabetes.
As Friedman’s work suggests, unpacking the myriad neural and biochemical pathways that create and regulate internal states could have major implications for human health.
Yet much work remains to be done.
Bargmann notes that, for the most part, scientists still do not know what initially triggers the internal states they have identified in various model animals.
Even more mysterious is how these states are maintained over time after the initial trigger has done its part. Neurons operate on millisecond timescales. Sometimes groups of neurons reinforce each other and stretch their activity to several seconds. But internal states persist on much longer timescales of minutes to hours.
“One of our biggest questions is how long-lasting internal states are generated and perpetuated,” Ruta says. “How do you maintain an arousal state that lasts for tens of minutes?” The answer may lie in the ability to look at larger and larger populations of neurons, observing activity across regions to gain a fuller view of the gears that keep a state running.
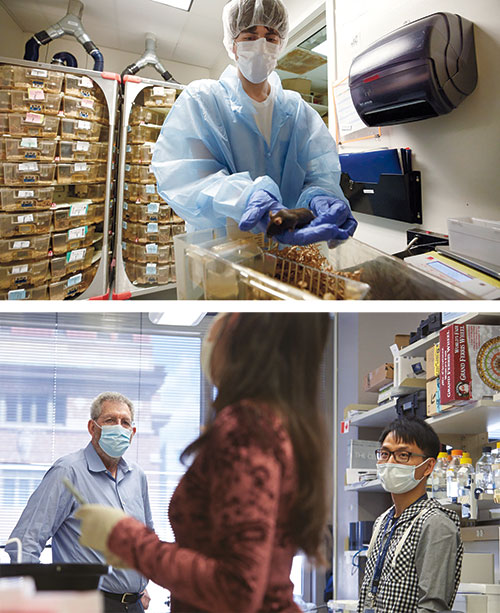
Getting at the underlying mechanisms that regulate the internal states could be tricky, says Michael W. Young, the Richard and Jeanne Fisher Professor. In 2017, Young shared the Nobel Prize in Physiology or Medicine for his work on biological clocks. Back in the 1980s, when he first began looking for genes that control Drosophila’s sleep-wake cycles, he could not have known that he would eventually discover a whole host of proteins that form a self-regulating clockwork in the fly’s cells. The discovery revealed biological principles that were later shown to apply to other animals, as well as plants, and control metabolism, development, and response to disease.
Those mechanisms establish what amounts to internal states of their own, driving behaviors that vary throughout a 24-hour cycle. And it turns out that the mechanisms of timekeeping are present in cells throughout the body, not just in the brain, suggesting a further level of complexity.
As our knowledge of the brain’s internal state mechanisms expands, it could have similarly sweeping implications, advancing our understanding of phenomena far beyond ravenous mice and lustful flies.
Bargmann, who in addition to her work at Rockefeller serves as head of science at the Chan Zuckerberg Initiative, points out that while mental health disorders such as anxiety and posttraumatic stress disorder impose a tremendous global health burden, neuroscientists are not nearly as good at understanding or remedying them as they could be.
Yet such disorders look very much like internal states run amok: a brain programmed to maintain a heightened state of alertness even when one is no longer necessary.
Similarly, Ruta suspects that decoding the neural basis for the regulation—and dysregulation—of internal states could have implications for attention deficit disorders. One of the principal functions of internal states, of course, is to focus our attention on and prioritize particular sensory inputs while filtering out others. And the same is true for autism spectrum disorder, which involves difficulty perceiving social cues, depression, anorexia, obsessive-compulsive disorder, and more.
Unraveling the factors that ordinarily initiate and terminate internal states could in time shed light on what causes them to be triggered too easily or to last longer than they should.
“We always have needs,” Ruta says. “Presumably, the brain is just continuously fluctuating from one state to the next.”