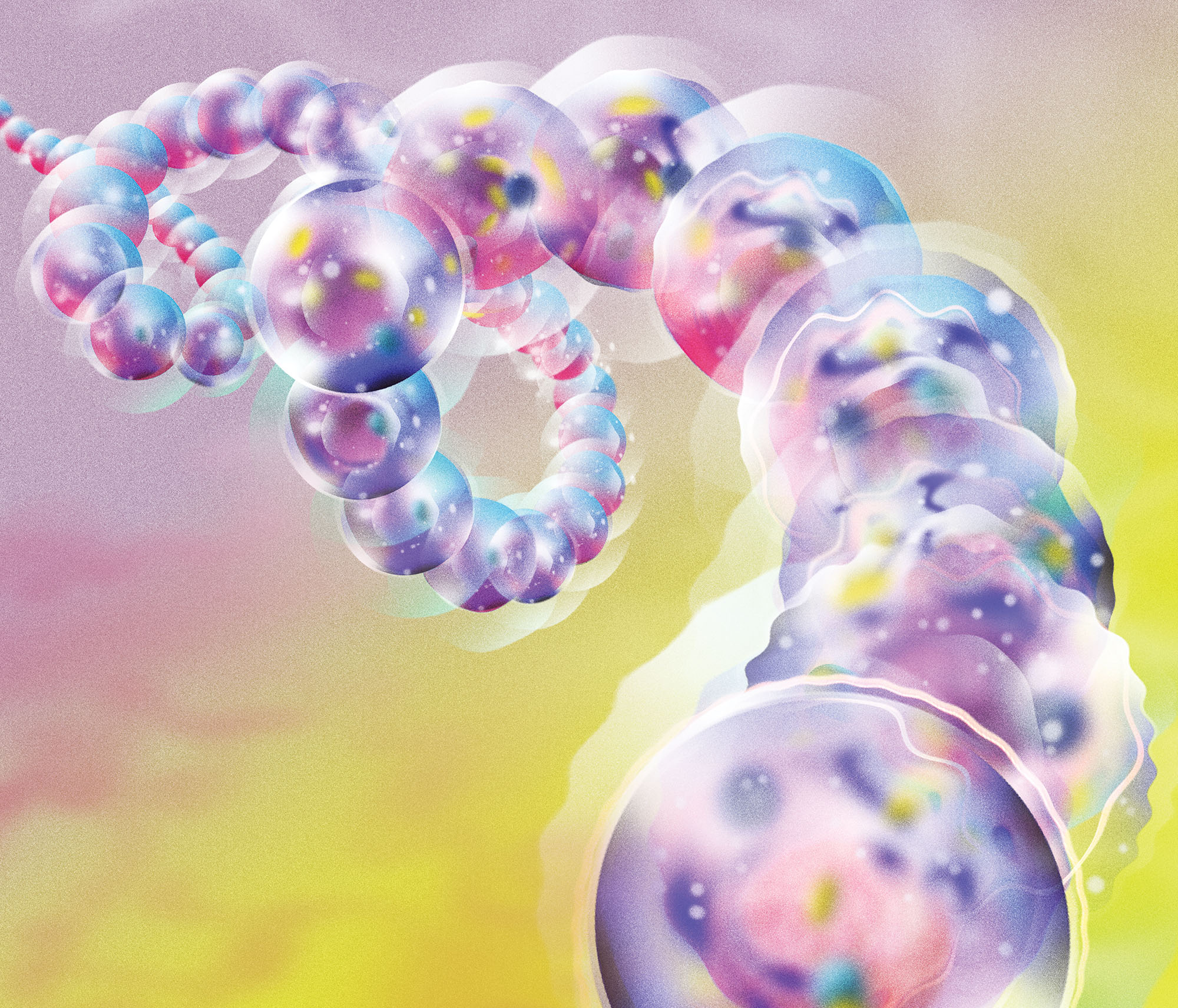
Mark Pernice
Feature
Stem Cells Are Growing Up
The controversy and hype have died down. The science is very much alive, creating new directions for discovery.
By Bahar Gholipour & Zachary VeilleuxPinched between his thumb and forefinger, on a round sliver of glass no bigger than a potato chip, Ali H. Brivanlou holds 10,000 human embryos arranged in a neat grid. It has the look of something vaguely electronic, like the inner workings of a smartphone. Yet under more natural circumstances, each of these embryos, a cluster of cells nearly invisible to the naked eye, would have the potential to create something unmistakably organic—a human being.
The lab’s incubators contain dozens of these robotically created wafers. The one Brivanlou is holding is just a few hours old, and over the next few days, it will undergo a course of biochemical treatments mimicking signals from the womb. It will then be placed under a specialized microscope, allowing Brivanlou and his colleagues to record the 10,000 embryos’ developmental journey.
But these embryos didn’t start out as fertilized eggs, and they will never develop into fetuses. The cell clusters, called synthetic or artificial embryos, are the descendants of human embryonic stem cell lines derived in Brivanlou’s lab 20 years ago.
“You can see them develop in front of your eyes,” he says. “If you watch them as they divide and begin to form into an organism, you will see the beauty inherent in nature, and you may learn some of its secrets. It is something that’s difficult to describe in words, but impossible to forget.”
Beneath the beauty, there is promise. What makes embryonic stem cells unique is their ability to morph into almost any type of cell in the body, like muscle cell, nerve cell, or blood cell. And because of this spectacular power, known as pluripotency, they’ve helped scientists forge countless new paths to discovery.
For example, stem cell research has delivered groundbreaking insights into the cryptic first stages of human development, and intriguing ideas about our evolutionary past. It has deepened our knowledge about the root causes of diseases like cancer, chronic inflammatory disorders, and degenerative conditions. Moreover, scientists are using stem cells as tools for a vast scope of work, from basic-science investigations of how the human genome is regulated to translational research seeking to pinpoint disease mechanisms or test novel drugs.
Today it’s hard to imagine where 21st-century science would be without stem cells, but their career as research subjects wasn’t always smooth.
It was at the start of the new millennium that scientists learned how to grow embryonic stem cells in the lab and nudge the cells to take on new identities. The research attracted headlines partly because of controversy around how the cells were obtained—typically from leftover embryos generated during in vitro fertilization treatment—and partly because they held promise for regenerative medicine, the idea of using stem cells to replace diseased cells or tissues in patients. Some advocates of the science made increasingly optimistic projections: With the right technology, it would soon be possible to grow whole organs in petri dishes. A patient’s worn-out liver, kidney, or heart could be swapped for a new one, like a set of tires.
“Claims were made about stem cells, particularly embryonic stem cells, that would clearly not be easy to fulfill,” says Brivanlou, who is Rockefeller’s Robert and Harriet Heilbrunn Professor.
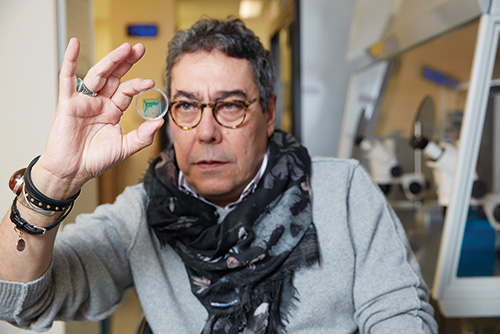
Indeed, working with human embryonic stem cells was challenging from the start, and remained difficult even after 2006, when new technologies allowed scientists to create so-called induced pluripotent stem cells (iPSCs) by reprogramming somatic cells such as skin cells into an embryonic-like state. The iPSCs behaved similarly to stem cells derived from embryos and promised a limitless supply of pluripotent cells for research and medical applications, but they came with their own peculiarities and safety concerns. Scientists also developed methods to guide iPSCs or human embryonic stem cells to differentiate into slightly more mature cells called progenitors, but attempts to push them further, toward full specialization, kept failing. There were multiple challenges, starting with the fact that no developing cell operates in isolation—to function normally, it must exchange signals with nearby cells and sometimes connect with nerves, arteries, and nonliving material in the tissue. It’s a puzzle with so many pieces that its solution may still be decades away.
50–70Number of times a normal human cell may divide before it starts to deteriorate.
∞Number of times a human stem cell may divide.
There were other hurdles, too. The creation of the first human embryonic stem cell line caused a cultural uproar so loud that it almost brought the science to a halt. Several European countries banned the creation of the cells, and in the United States, then president George W. Bush cut off the field’s access to government funding. Scientists had to secure private funding for work involving embryonic stem cells and, to comply with federal regulations, erect physical barriers in their labs to separate privately funded work on stem cells from government-funded research.
It was in such a cordoned-off corner that Brivanlou’s team began studying natural human embryos and deriving cell lines from them. They also developed a platform that made it possible to grow the embryos in petri dishes, even past day seven, when normal embryos need a uterus to survive. For the first time, they could watch the transformations taking place during the second week of human embryogenesis, up until around day 14. This is when a milestone called symmetry breaking occurs—characterized by the emergence of the body’s three axes (head to foot, front to back, left to right).
To observe later developmental stages, Brivanlou’s lab and Rockefeller physicist Eric Siggia created synthetic human embryos growing as self-organizing 3D cultures. These embryo models mimic the geometry of natural embryos and can be induced to undergo symmetry breaking, giving researchers a way to study the biological events occurring in the third week of development. They have also provided some of the most striking pictures of early human life ever seen.
Synthetic embryos are not only answering questions about human development, however. Brivanlou’s lab and others are also using them to study medical problems, including limitations in technologies to help couples conceive. In a study last year, for example, the team identified an avoidable bottleneck in in vitro fertilization procedures, a finding that “may change the way IVF has been done for the past two decades,” Brivanlou says, “and make it succeed for many more people.”
Currently, only a small fraction of embryos generated in fertility clinics are used for transfer to the uterus. Most are deemed deficient, flagged by a test detecting an abnormal number of chromosomes. But the team found that these supposedly nonviable embryos often self-correct as they grow, in a complex process that eliminates cells with irregular chromosome numbers from the fetus. These results are in line with earlier observations that when such abnormal embryos are implanted in a prospective parent, they survive just as often as embryos that test normal and produce healthy babies.
“These embryos are viable and should no longer be discarded,” Brivanlou says.
Moreover, the synthetic embryos have created unprecedented opportunities for studying disease mechanisms and testing new drugs. One striking example is the lab’s use of the technology to study Huntington’s disease. This inherited neurodegenerative disorder was long thought to start in middle age, when symptoms typically emerge. But the researchers found that earlier signs of Huntington’s arise before birth, in the first two weeks of embryonic development. “This suggests that what we’ve long thought of as an age-related condition may in fact be a developmental disease,” Brivanlou says.
Another technology that emerged from stem cell research is the organoid. From their human embryonic stem cell lines, Brivanlou and his colleagues created brain organoids—tiny 3D cultures of embryonic neural tissue for studying Huntington’s and screening for novel drugs. And when the pandemic hit, they created other organoids that model embryonic lung tissue, providing a system to investigate how SARS-CoV-2 damages airways and alveoli in people with severe COVID.
“This technology really opens a door to identifying the mechanisms by which organs like the brain or lungs normally develop, understanding how they go awry in disease, and testing drugs that set these mechanisms back on the right course,” says Brivanlou.
A century and a half of stem-cell science
Scroll for more →
-
1868
German biologist Ernst Haeckel coins the term stamzelle (stem cell in German) describing the hypothetical ancestral cell from which all multicellular organisms evolved.
-
1892
German scientists Theodor Boveri and Valentin Häcker pronounce the stem cell the mother cell of the germ line, based on the theory that there is a common cell capable of self-renewal and differentiation.
-
1958
The first allogeneic bone marrow transplant is performed in France, on survivors of a nuclear accident. Later, scientists will learn that stem cells are the key component of the lifesaving therapy.
-
1964
Canadian biologists Ernest McCulloch and James Till provide the first experimental evidence of the hematopoietic stem cell from which various types of blood cells originate.
-
1981
British scientists Martin Evans and Matthew Kaufman and American biologist Gail Martin isolate and culture the first embryonic stem cells from mouse embryos.
-
1983
Howard Green, an American physician-scientist, successfully treats burn victims with skin grafts derived from epidermal stem cells.
-
1988
The U.S. government places a moratorium on federally funded research on fetal tissue transplantation. Over the next 30 years, similar restrictions will be repeatedly repealed and reenacted.
-
1998
A team led by American developmental biologist James Thomson isolates the first batch of human embryonic stem cells.
-
2006
A Japanese group led by stem-cell scientist Shinya Yamanaka creates induced pluripotent stem cells by reprogramming adult cells to an embryonic state.
-
2009
Stem cell lines derived at Rockefeller are among the first to be included in a National Institutes of Health repository.
-
2009
The first human trial of an embryonic stem cell-based therapy is cleared by the FDA to treat patients with severe spinal cord injuries.
-
2009
A team led by Dutch molecular geneticist Hans Clevers creates the first organoids from adult stem cells of the intestine.
-
2011
Elaine Fuchs identifies cancer stem cells of squamous cell carcinoma and shows that these cells make tumors more aggressive.
-
2014
Ali H. Brivanlou develops a microchip-based system to grow synthetic human embryos in culture.
Learn more about these milestones in the story.
In the 1970s, a series of botched experiments led Howard Green, a researcher at the Massachusetts Institute of Technology, to a surprise discovery. While unsuccessfully trying to replicate a rare cancer in a petri dish, Green noticed that the tumor-derived cells were forming structures resembling the outer layer of the skin, or epidermis. This “test-tube skin,” as he called it, was so similar to real skin that it could be used for skin grafting. In an oft-cited procedure in 1983, Green and others regenerated massive amounts of epidermis and saved the lives of two boys whose bodies were covered with third-degree burns. But Green didn’t know why his cultured cells could magically generate new tissue. What was going on inside that dish?
50–150Number of cells in an embryo five days after fertilization.
Eventually, he figured it out: Some of the cells had the capacity to divide over and over without specializing. Without these cells, now known as adult stem cells, no epidermal structures would grow.
Adult stem cells can be found in virtually all the body’s organs, where they live in localized homes called niches and self-generate to replenish the surrounding tissue. If it weren’t for adult stem cells, these organs would wither and we wouldn’t live long.
Elaine Fuchs was in graduate school when a lecture by Green upended her plans. Well on her way to becoming a microbiologist, she was so intrigued by the new discoveries that she switched gears and joined Green’s lab as a postdoc. Today, four decades later, she continues to study epidermal stem cells, which have guided her to numerous discoveries in far-ranging fields, from inflammation to cancer.
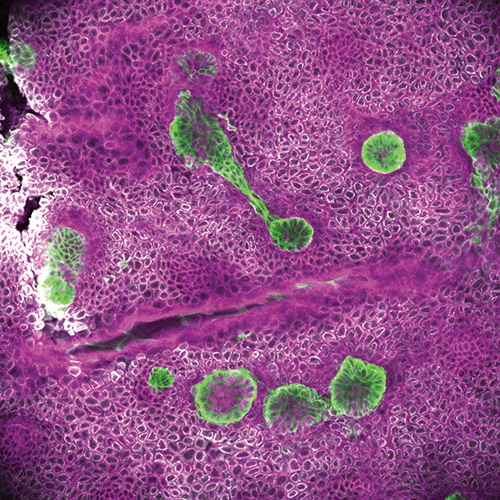
Fuchs realized early on that to understand the rejuvenating power of stem cells, she needed to study not only the cells themselves but also their niches, and the dynamic interactions taking place among stem cells, their neighbors, and their local environment. “A key discovery was that adult stem cells require other cells in their surroundings to be able to behave properly,” she says. “Many recent breakthroughs in the field—like organoid cultures, for example—are based on the knowledge that stem cells are not operating in a vacuum.”
Because stem cells continually exchange signals with their surroundings, Fuchs adds, all efforts to grow tissue in a dish begin with decoding the signals those cells use to communicate.
In the skin, specialized stem cells make the epidermis, hair follicles, and sweat glands; and in other organs like the intestines and lungs, different epithelial stem cell types regenerate similar absorptive layers. Bit by bit, Fuchs’s lab is piecing together what goes on in the niches of these different stem cells, pinpointing the specialized microenvironments that nourish and guide them, and how those environments change in injury, inflammation, and cancer.
“Many recent breakthroughs are based on the knowledge that stem cells are not operating in a vacuum.”
“In the skin, there are 65 different cell types, and we believe a good number of them interact with stem cell niches in various ways and at different times,” Fuchs says. Neurons, fibroblasts, adipocytes, muscle cells, and immune cells all communicate with skin stem cells to do the right thing at the right time. And recently, the lab discovered that the lymphatic vasculature wrapping around the niches synchronizes the activities of stem cells across a tissue. The dynamic interplay between stem cells and their niche is at the heart of the skin’s routine maintenance and its ability to repair wounds, Fuchs says, and it also plays a role in many medical conditions—enough to keep her lab perpetually busy.
“How are stem cells suddenly called into action to regenerate tissue damaged by injury?” she says. “How do they defend against pathogens and cope with infections and inflammatory stress? And why do they sometimes malfunction?”
In eavesdropping on stem cells in their niche, the lab has made several surprising discoveries. Among them is the fact that many chronic conditions traditionally considered immune disorders—including psoriasis, atopic dermatitis, inflammatory bowel disease, and asthma—might be the result not of a malfunctioning immune system but rather what happens when epithelial stem cells lose the ability to mount a robust barrier against pathogens or when they miscommunicate with the immune system. Moreover, Fuchs and her coworkers suspect that chronic inflammatory diseases may occur because stem cells harbor memories of past inflammatory episodes, causing them to react too quickly to a new trigger.
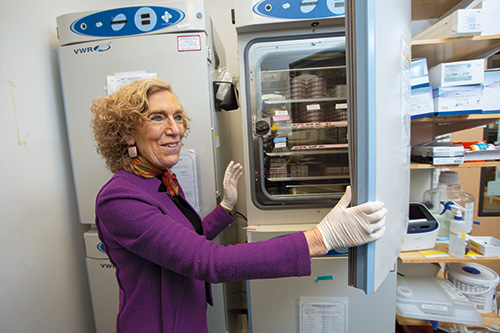
It was Fuchs and her colleagues who discovered in 2017 that stem cells draw on their memories in responding to threats—a process similar to immune cells’ ability to remember a pathogen and respond more quickly on subsequent encounters. It’s generally a useful adaptive mechanism that strengthens the body’s defenses throughout life. The problem, Fuchs explains, is that repeated assaults might cause the cells to overreact to irritants or pathogens, leading to runaway inflammation that may manifest as rashes, pain, and other symptoms typically seen in these disorders. And her research suggests that stem cell memories are long-lasting: In a lab mouse, they can persist for up to six months, the equivalent of about five human years.
Can these memories be erased? Fuchs thinks so, and her team is exploring this possibility. Ultimately, the researchers hope that their work will lead to the development of new treatments for chronic inflammatory conditions to replace current immunosuppressive drugs, which have undesirable side effects and are not always effective.
1Number of epidermal stem cells needed to generate enough skin to cover the adult body.
>200Number of cell types in the human body.
There are other circumstances in which adult stem cells trigger disease instead of healing and protecting their native tissue. One is metastatic squamous cell carcinoma, a highly aggressive type of skin cancer that Fuchs and her coworkers have studied for many years.
Like most of the body’s tissues, healthy skin epithelium contains many mutant cells, which is usually not a problem. When a mutant shows up, neighboring cells will keep it in check by curbing its proliferation, for example—and after a day or two, the cell will die. But if the mutant happens to be a stem cell, which is the case in squamous cell carcinoma, the scenario becomes far more dangerous. Because it can self-renew indefinitely, the stem cell will hold on to all the mutations it has gained in the past, building a vast repertoire of genetic errors and improving its chances of dodging the intrusive neighbors.
How does this cell dupe nearby cells, and how does it cut itself loose from its locale of origin to leak into the bloodstream and metastasize? “The epithelial stem cell has to manage to survive in order to give rise to cancer, and to do that it needs to change the way it communicates with other cells,” Fuchs says.
65Number of cell types in the skin.
>1MNumber of stem-cell transplants performed to regenerate blood cells in patients.
Recently, her team found that squamous cell carcinoma cells exit their native nook by giving self-serving instructions to neighboring epithelial stem cells that maintain the surrounding epithelium. “Epithelial stem cells normally control the tissue architecture,” Fuchs explains. “But when a mutant cell induces them to change their gene-expression program, they begin to lose control of the mechanical properties needed to keep the tissue supple and healthy.”
She and her coworkers found that the mutant cell can create an escape route by generating stiffer surrounding tissue. As a result, mechanical forces build up, allowing the mutant to break through and pierce into deeper layers of the skin, from where it can spread further throughout the body via capillaries. More benign skin cancers such as basal cell carcinomas, on the other hand, produce less rigid cell structures and are more likely to stay put.
The lab is now working to pinpoint which of the many mutations found in squamous cell carcinomas are responsible for hijacking the normal ability of epithelial stem cells to proliferate and heal wounds. “Figuring this out will get us much closer to understanding how the disease advances and develop effective interventions,” says Fuchs, the Rebecca C. Lancefield Professor.
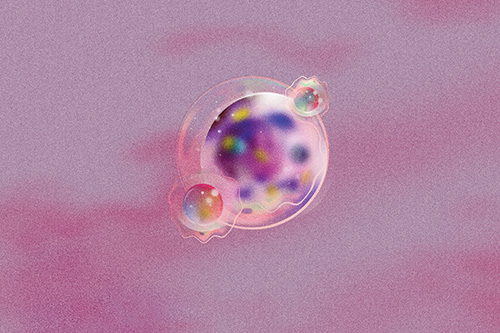
Like Fuchs, C. David Allis followed the science where it led him—and became a cancer biologist along the way. A pioneer in the study of epigenetics, he began working in stem cells because they provide a convenient model, a cell-based system for studying an organism’s entire genome in action. While specialized cells are more obscure—they’ve typically closed off all the genes they don’t need—stem cells are a blank slate. Every part of their genome is flexible, allowing scientists to explore a vast universe of epigenetic programming taking place in development, dementia, depression, or your biological context of choice.
With his coworkers, Allis, who is Rockefeller’s Joy and Jack Fishman Professor, has discovered several epigenetic mechanisms that normally keep stem cells in check but may be disrupted in cancer. Yadira Soto-Feliciano, a former postdoc in the lab who recently became an assistant professor at MIT, says this chain of events happens in pediatric cancers in particular.
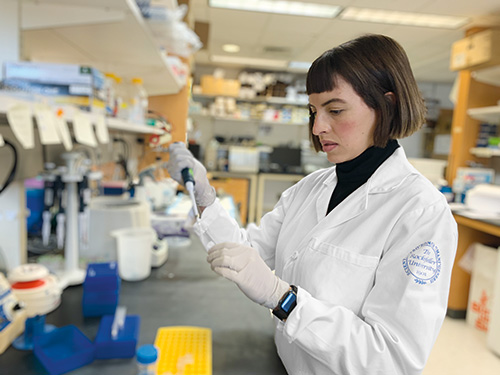
Why pediatric cancers? And why are few adult cancers caused by these epigenetic mishaps? To answer these questions, Soto-Feliciano wants to know what else distinguishes a young person’s cancer from that of an adult. Focusing on childhood blood cancers, she is pinpointing the mechanisms that mess up stem cells’ epigenetic programming in the first place.
Recently, she and Allis found a clue. They discovered that in pediatric leukemia, the initial disease culprit is a molecular machine that normally prevents malignant transformation by quieting certain genes in stem cells of the blood. A DNA rearrangement creates a riotous version of the machine that switches the genes on rather than off. Cancer ensues.
“The cells begin to proliferate rapidly, but without actually reaching their final form,” Soto-Feliciano says. “They become frozen in a stem cell-like state and can’t mature into blood cells.”
In her lab at MIT, she’s looking for a way to free these trapped stem cells and help them grow out of their “stemness”; and she’s exploring the possibility of developing new drugs based on that principle. The task may be accomplished by targeting the mutant machine with small molecules (several such molecules are currently in clinical trials for leukemia). But there is an additional layer of complexity: Soto-Feliciano has found that leukemia cells thrive not just by turning on growth-fueling genes, but also by shutting down other genes that normally suppress growth. She is now testing a combination therapy that, in addition to neutralizing the growth-fueling mutant, also switches suppressor genes back on, finishing off the cancerous cell once and for all.
“Ultimately, the disease is a fight between two types of genes,” she explains, “those that promote cancer by making stem cells unable to differentiate, and those that suppress tumor growth by making the stem cells proceed normally through development. An ideal cancer therapy will address both ends of that equation.”