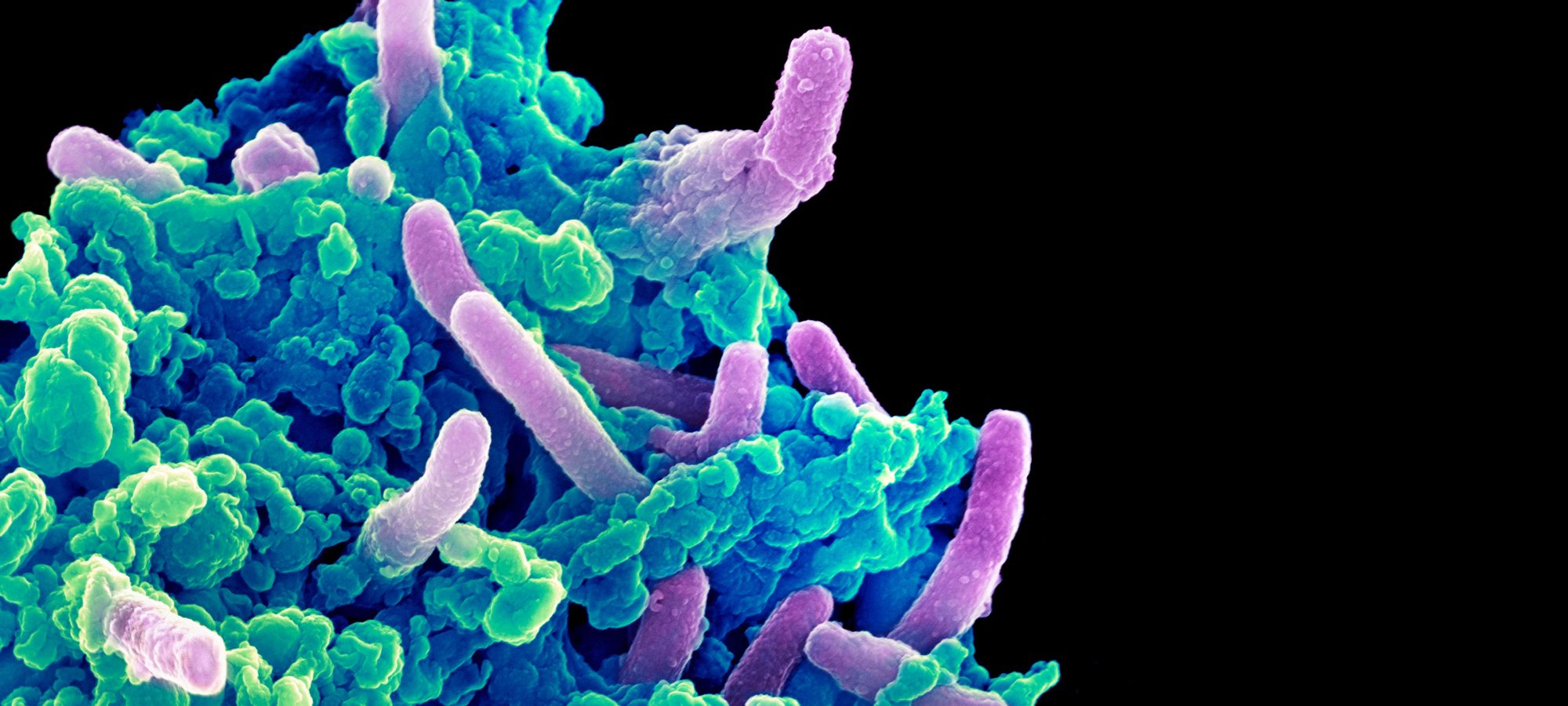
Feature
TB is changing. So is science.
Tuberculosis keeps reinventing itself. As drug-resistant strains spread across the globe, it’s becoming increasingly harder to wipe out. But researchers, too, are adjusting—and they’re better positioned than ever to attack one of humanity’s most ancient health problems.
By Alison McCook and Eva KieslerFor a time when Elizabeth Campbell was in her early 20s, any sweat that trickled down her brow or armpits was an eye-catching bright orange. “I felt quite self-conscious in the gym,” she recalls.
Orange perspiration is a known side effect of a drug used to treat and prevent tuberculosis, one of the world’s most deadly diseases. When Campbell started graduate school, a decade after she and her family had immigrated to the United States from their native Guyana, she was required to take the drug for six months because telltale antibodies had been detected in a blood test. (The antibodies were most likely due to her childhood vaccinations, not to an active infection, but with TB it’s best not to take chances.) Six months is a long time to be on a strong medication you almost surely don’t need, but Campbell swallowed her neon pills every day, without complaint.
“I come from a place where, if you don’t take your antibiotics, you die,” she says.
Across the developing world, Guyana included, TB remains a scourge, and yet those of us with access to good health care, hygiene, and nutrition pay little attention to it. TB kills more people than any other infectious agent, even malaria and HIV. In 2017, 10 million people became sick with TB, and 1.6 million died.
Outmaneuvering TB will involve designing drugs that act faster. The sheer duration of existing therapy has likely contributed to the drug-resistance problem.
Antibiotic resistance is one of the reasons the disease is out of control. Campbell’s orange drug, rifampicin, along with a few others, are currently the best treatment available for TB, but several M. tuberculosis strains have evolved techniques to survive them. It’s an escalating problem that public health experts warn might soon bring on a full-blown disaster—a world in which antibiotics offer no protection whatsoever and people everywhere become infected—and it has sent researchers like Campbell, a research associate professor, and Jeremy Rock, a new assistant professor, scrambling to come up with better drugs.
It’s often a struggle. For decades, scientists’ hands have been tied by a lack of adequate tools. For a variety of reasons, M. tuberculosis is difficult to study; the techniques that have worked for scientists focusing on other infectious diseases have failed with TB. But this is starting to change.
Although the pandemic remains fierce, Campbell, Rock, and their colleagues believe the TB field is poised to turn a corner.
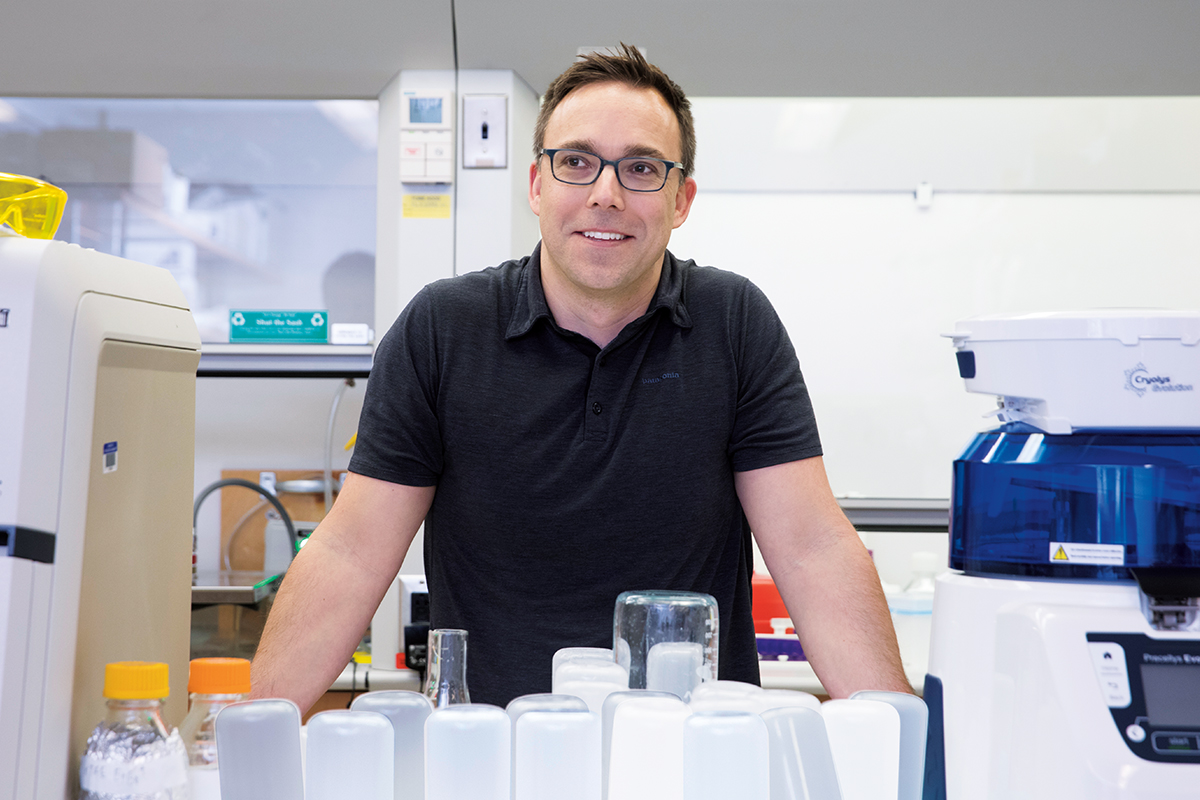
The first time Rock held a flask filled with enough bacteria to infect almost the entire world with TB, he felt a little nervous. He was a postdoc at Harvard University, working in the labs of Sarah Fortune and Eric Rubin, and brand new to the study of deadly infections; his former research subjects had been harmless, easygoing yeasts.
Rock’s plan as a newcomer to the field was to search the M. tuberculosis genome for genes the microbe cannot live without. He hoped those genes would lead him to new targets for future drugs. But many people were doing similar searches. “The work was very difficult and slow,” Rock says. “If you wanted to manipulate genes to figure out their function, you had to knock out each gene one by one.” If you were unlucky, targeting even a single gene could involve years of trial and error.
Rock was sure there had to be a more efficient, more systematic way to comb through M. tuberculosis DNA. If only he could get the right tools, he felt optimistic that the search would turn up something useful.
The first few roadblocks he encountered were anticipated. One was the incredibly slow growth rate of M. tuberculosis, which makes experiments very time-consuming. (It may also be a reason the pathogen is so effective at spreading—it is able to lurk in the body for years before symptoms develop, so many infected people don’t even know they have it. Public health experts estimate that up to one-quarter of the global population is currently infected with this latent form).
Another well-known hurdle is the infectious prowess of M. tuberculosis, among the deadliest microbes known to prey on humans. In the real world, a cough, sentence, or song from an infected person can put you at risk; to handle it in the lab you need specialized, expensive containment gear.
But there were novel obstacles as well. Rock believed that if anything could energize research on TB, it was CRISPR interference, a technology that makes it possible to easily turn off genes and study the consequences. The method can be scaled up to allow for very broad searches, with lots of genes being explored side by side.
CRISPR interference had already begun to revolutionize biology; scientists working on everything from maize to mice were getting in on it. But when Rock tried it on his finicky but deadly bacteria, it didn’t work. Either CRISPR didn’t knock genes down properly, or it killed the cells before any data could be collected. He tweaked his strategy in every conceivable way before he finally gave up. “It took us two years to accept that a tool that works great for everyone else didn’t work well for us,” Rock says.
And so, he began the laborious work of building his own tool.
One common method to edit an organism’s genome takes advantage of two collaborating bacterial proteins: CRISPR (hence the name “CRISPR interference”) and Cas9. In nature, CRISPR-Cas9 serves as a kind of immune system for bacteria known as Streptococcus pyogenes, but Rock knew there are other microorganisms with different versions of Cas9. What if one of these Cas9 cousins just happened to be more suitable for M. tuberculosis?
Rock tried 11 different systems before stumbling upon Streptococcus thermophilus, a bacterium commonly used to ferment dairy products. “I’ll never forget watching the results come out in real time,” recalls Rock. “I almost didn’t believe it, given how many failures we’d had up to that point.”
Last year, after completing his postdoc at Harvard, Rock launched his own lab at Rockefeller where he is now “carpet bombing the TB genome” with his custom-built CRISPR interference technology. The wide-scale approach will not only help the lab identify the pathogen’s weakest spots more quickly, Rock says, but will also allow them to study the relationships between genes—information that is highly relevant for developing combination or “cocktail” therapies.
“TB is always going to have to be treated with a combination of drugs,” Rock says. “Even now, you need to take a four-drug combination cocktail to curb the infection. So we’re very interested in learning how to build stronger drug combinations. For example, can we exploit synergistic interactions between genes by pairing drugs that enhance each other’s effects?”
“Can we exploit interactions between genes by pairing drugs that enhance each other’s effects?”
Seven years into his research with TB, and one year after starting his own lab, handling a deadly pathogen doesn’t scare Rock anymore, and he’s become quite comfortable with the Tyvek suits, HEPA masks, and double gloves required to work with it, even if it gets hot in the summer.
Although he is currently conducting his work in a highly secure facility in the lab of Carl F. Nathan, chair of microbiology at Weill Cornell Medicine, Rock is also helping design a new high-biosafety facility on Rockefeller’s campus, set to open this year. It’s an investment in the future of tuberculosis research, setting him and other scientists up for a vast range of experiments.
Already, Rock’s lab has identified genes that may have the markings of a drug target, and he hopes to soon initiate functional studies of those genes in TB-infected human cells or mice. Although it’s promising work, and enough to keep Rock and his lab members busy for years to come, he’s quick to point out that his approach is but one piece in a much larger puzzle. Having thwarted humanity for many centuries, M. tuberculosis won’t give up without a fight.
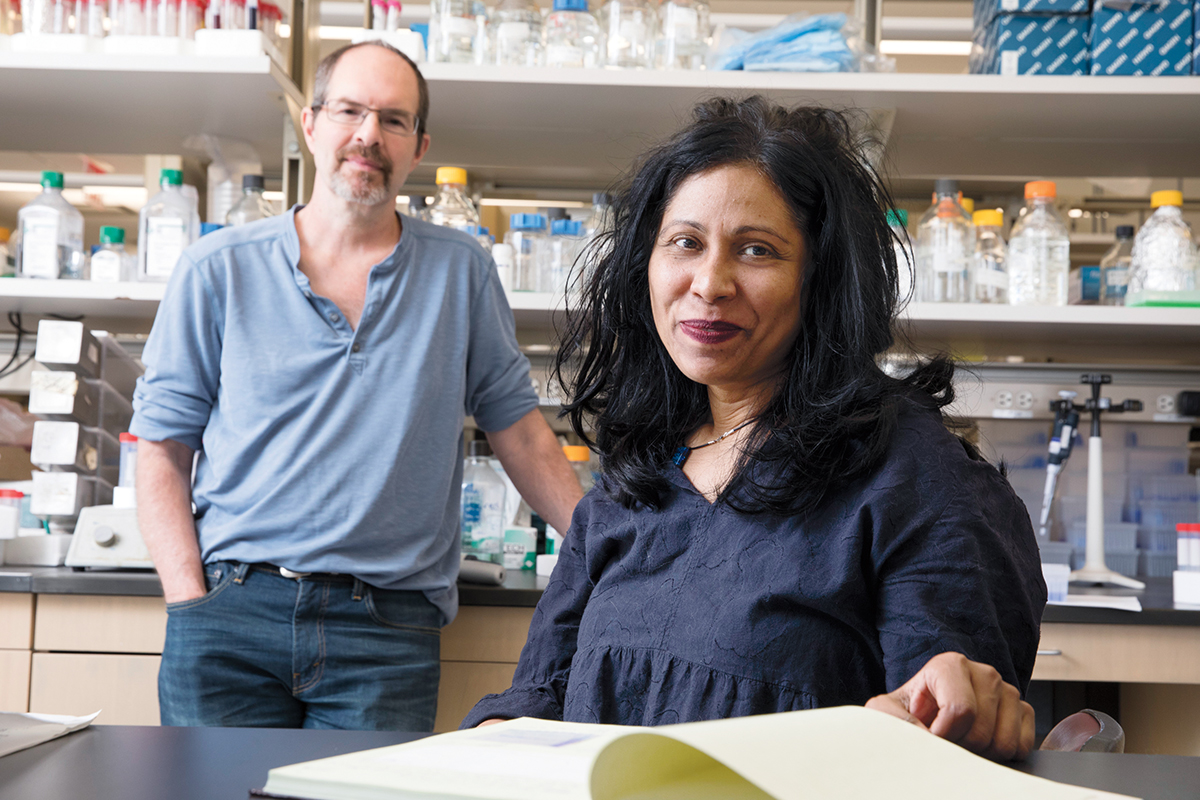
Campbell and her colleagues are attacking the disease from a different angle. Experts in structural biology—a school of science that solves problems by drawing precise three-dimensional maps of interacting molecules, atom by atom—she and her team are looking to old drugs for new ideas. In particular, they are conducting extraordinarily detailed investigations of how rifampicin interacts with its target, gaining information that might prove useful in multiple ways. By elucidating how M. tuberculosis interacts with the drugs currently in use, Campbell hopes to create opportunities for medicinal chemists to either troubleshoot and improve these drugs, or design new ones that could be used in combination with existing therapies.
Campbell and her collaborator Seth A. Darst, the Jack Fishman Professor, share an interest in TB but came to it from somewhat different perspectives.
A biochemist, Darst has devoted most of his career to understanding an enzyme called RNA polymerase, or RNAP (pronounced “ar-nap”). The enzyme is in charge of one of life’s most basic operations: It reads DNA to make RNA, the blueprint for proteins, in a feat known as gene transcription. Every cell on the planet depends on it.
In other words, RNAP ranks high on the list of evolution’s biggest innovations, and Darst has never had a shortage of reasons to be interested in it. But RNAP also happens to be the target of several antibiotics, including rifampicin.
Campbell, on the other hand, is trained in microbial pathogenesis and witnessed people dying from infectious diseases as a child. When she joined Darst’s lab in the early 2000s, her main goal was to understand the therapeutic potential of RNAP. But when the two started to ask questions about RNAP in relation to TB, they soon realized that answers would be very hard to obtain.
At the time, the only way to visualize how rifampicin acts on RNAP was to coax the embracing molecules into a crystal, then bombard that crystal with a beam of x-rays. However, as you might expect from a pathogen with a proud legacy of frustrating scientists, M. tuberculosis’s version of RNAP turned out to be notoriously difficult to crystallize.
For years, the pair tried work-arounds. They were able to glean several details from RNAP present in a similar bacteria, Thermus aquaticus, which also responds to rifampicin. They learned that rifampicin disables RNAP by latching on to a structure deep inside one of the enzyme’s elaborate pockets and throws a wrench into the machinery that transcribes DNA into RNA. As the enzyme glides along a DNA strand, it produces an ever-lengthening tail of fresh RNA—and sooner or later, this tail bumps into the antibiotic and falls off, preventing further transcription.
They also discovered that a handful of M. tuberculosis mutations that were known to cause rifampicin resistance all appeared to make tiny structural changes to the very site of RNAP where the rifampicin normally inserts itself, presumably preventing the drug molecule from docking. “This was a big step toward understanding both antibiotic resistance and how RNAP works in general,” says Darst. “When we understand where the antibiotics bind, and how they work, that tells us a lot about the enzyme’s basic function.”
A few years ago, Darst and Campbell got their first good look at the M. tuberculosis RNAP, thanks to new technology that circumvents the crystallization process. With cryo-electron microscopy, or cryo-EM, which uses electrons rather than x-rays, and allows scientists to study protein structures without crystallization in their native states, Darst and Campbell can now visualize RNAP from M. tuberculosis itself, in more exquisite detail than ever before.
But even this breakthrough didn’t come easily. Cryo-EM requires a purified sample of M. tuberculosis RNAP. And that’s when the arguments started.
Campbell recounts how she, working with a research assistant in the lab, purified batch after batch of the RNAP, all showing zero activity—the enzyme was virtually dead. “When we showed our results to Seth, he told us we simply didn’t know how to purify RNA polymerase,” Campbell laughs. “And so we went back to the bench. We couldn’t get it to work.”
For months, the two debated what was going wrong. Darst, with his deep understanding of RNAP, claimed Campbell needed go back to basic biochemistry textbooks to figure out the glitch, while Campbell insisted that the M. tuberculosis RNAP wasn’t working the way it does in other organisms. Gradually, he began to realize she may be right.
A breakthrough came when collaborators Michael Glickman and Christina Stallings at the Memorial Sloan Kettering Cancer Center sent the lab a sample of a protein called CarD, which they had discovered was essential for M. tuberculosis survival. On a whim, the Rockefeller scientists poured some CarD on their biochemically impotent RNAP, and—lo and behold—it came alive.
Since that moment, new insights have been flooding in, and show no signs of slowing down. Campbell and Darst have discovered that, unlike other bacteria, RNAP from M. tuberculosis requires two helper proteins—CarD and another cofactor called RbpA—to function properly.
If the discovery of four-letter cofactors sounds esoteric, it also happens to be news we could use. For example, drugs already exist that bind to RbpA when it is bound to RNAP, potentiating the drug’s activity. One of them might boost the effectiveness of rifampicin against TB, the scientists speculate, or perhaps new drugs could be developed that thwart CarD. An exciting aspect of this idea, says Campbell, is that drugs targeting such cofactors would specifically kill M. tuberculosis without disrupting the body’s “good” microbes—like those of the gut—and may therefore be less likely to cause side effects.
As their TB work is making Campbell and Darst busier than ever, their teenage daughters—did we mention they are married?—have had to lay some ground rules, like taking breaks from talking science at their family dinners. (One of the girls, who once captured her parents quarreling about purification protocols on Snapchat, complains that “other couples fight about money, but all you guys ever fight about is RNA polymerase.”)
In January, the pair published cryo-EM results in Nature showing snapshots of the M. tuberculosis RNAP in action, just as it’s separating the two strands of DNA to make RNA. Their findings revealed just how slowly the enzyme works—explaining, perhaps, why this pathogen grows at such a leisurely pace compared with other bacteria. They’re hoping this insight might help explain other aspects of the infection, including why the bacteria lies dormant in so many patients.
There’s still a long way to go, but every day more of the picture comes into focus. Campbell and Darst are starting to team up with Rock, as well as with Sean F. Brady, Rockefeller’s Evnin Professor, who is looking for natural products that could be useful in treating TB and other infections (read more about Brady’s research in “Terrestrial treasures”). They are hoping their work will yield new strategies to outsmart the bacterium’s tendency to acquire drug resistance, including, possibly, new faster-acting drugs.
This is important, Campbell explains, because the sheer duration of existing therapies—six months in her own case, often years for people with antibiotic-resistant strains—has likely contributed to the emergence of drug-resistant strains.
“I won’t make a claim that our work is going to cure TB,” Campbell says, “since that will require a combination of efforts and approaches. But here at Rockefeller, a number of scientists are taking different approaches to the same problem. And working together, we can really make some headway.”