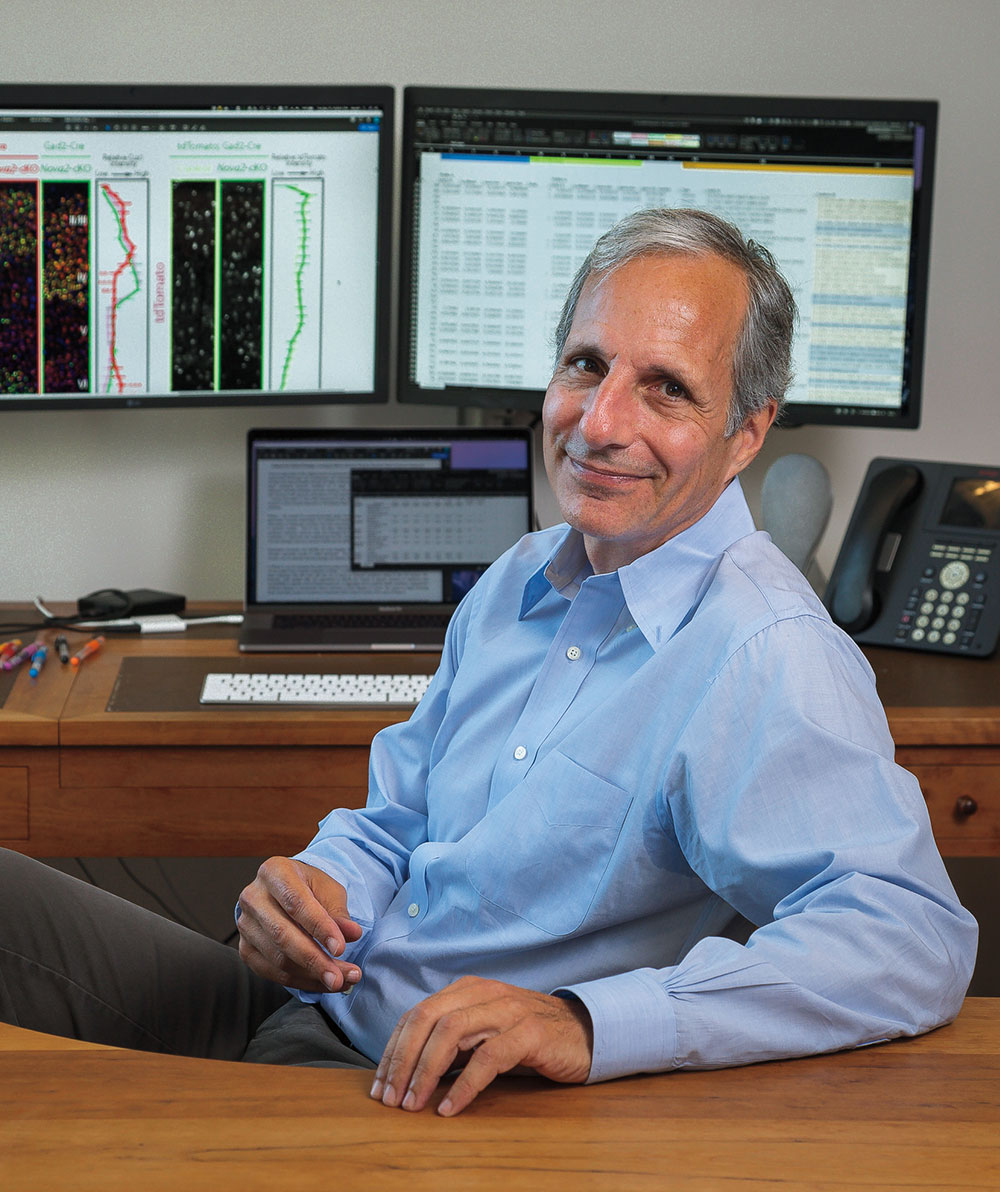
Interview
The world’s most powerful molecule has a PR problem
With Robert B. Darnell
By Eva Kiesler and Caitlin ShureWith its iconic shape and voluminous genetic script, DNA is the darling of nucleic acids. Since 1944, when Rockefeller scientists first identified it as the substance of hereditary information in cells, DNA has been center stage in biology, heralded as the blueprint of everything that organisms are or have the power to become. So popular is the splendid spiral that it has come to be visual shorthand for life itself, embodied in everything from fine art to postage stamps and $10 gift-shop bracelets.
Yet DNA isn’t necessarily the most interesting of molecules. To realize its potential, which usually means to make proteins, it must first be copied into fragments of a less famous molecule: ribonucleic acid, or RNA. RNA’s main job is to messenger DNA’s instructions to the cellular machinery that makes proteins.
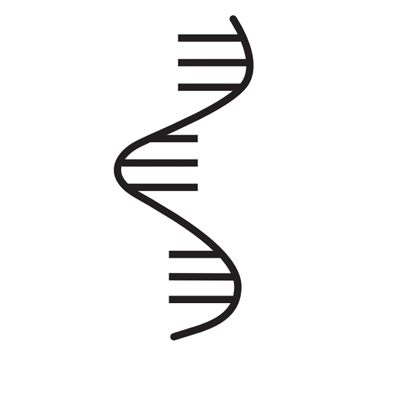
Data
As much as five percent of the weight of a human cell is RNA.
But RNA is not merely a messenger. In recent decades, researchers have been surprised to discover how many different types of RNA there are, and the myriad ways in which they help cells manage their basic operations. RNA is even believed to have instigated life on Earth, long before DNA and proteins came into the picture. And it is turning out to be a worthy subject for clinical science. For example, the level of certain RNAs in a patient’s tissue might help predict disease outcomes, offer important insight into the nature of the disease, and in some cases even open doors to new treatments.
Clinician-scientist Robert B. Darnell, Rockefeller’s Robert and Harriet Heilbrunn Professor and the founding director of the New York Genome Center, has spent decades investigating RNA and the proteins that help regulate it in the context of brain diseases and cancer. We asked him to tell us more about the mysterious molecule to which we, along with 8.7 million other species, owe our existence.
What should people know about RNA?
All your cells have the same DNA, which is basically an inventory of all the things our cells are theoretically able to do. RNA, on the other hand, is customized to the situation. Its job is to determine what any given cell actually ends up doing at a specific time or under specific circumstances. When we talk about gene expression, the process by which a piece of DNA gets activated to produce a protein, we’re often really talking about RNA. There’s a whole layer of regulation that alters both the quality and the quantity of that protein, much of which involves RNA.
“RNAs are the driving force of biological complexity.”
Furthermore, many genes are modular, meaning they can be assembled in different ways at the RNA level. The result of this process is that a single gene may produce not just one, but hundreds of versions of a protein that differ in their structure and function. It’s a way in which evolution has allowed organisms to become increasingly sophisticated. Generally speaking, bacteria make as many proteins as they have genes, but humans, thanks to alternative splicing, make many more. RNAs are the driving force of our biological complexity.
But RNA gets really fascinating when we consider its feat as the very origin of life on Earth. In a likely scenario, the earliest life form was a membrane surrounding a piece of RNA capable of doing two things: carrying its own genetic recipe and acting as an enzyme to make new copies of that recipe—in other words, it could replicate itself.
If RNA came first, what was the purpose of DNA?
At some point, replicating RNAs needed a more reliable system to safeguard their genetic content from damage. Many scientists believe that an early cell solved this problem by making backup copies of the RNA sequence in the form of DNA, which is more robust. That’s how cells ended up with two versions of the genetic code: a DNA version that ensured data safety and an RNA version that allowed the data to be used in a flexible manner.
Another evolutionary breakthrough, of course, was the addition of proteins. Early organisms that used RNA to make proteins had a big advantage over those that didn’t. RNA is made up of only four basic building blocks while proteins are built from 20, which means proteins can assume more elegant and functional shapes.
Back to the present—how does RNA influence complex life process like those involved in human cognition?
The human brain is the most complex biological system we know. It consists of many billions of cells, and each can make thousands of connections with other cells, with millions of connections being modified every second. The brain’s ability to produce highly sophisticated functions like cognition is believed, at least in part, to result from mind-boggling intricacy at the RNA level.
It’s not uncommon for genes expressed in the brain to produce hundreds of protein variants; and there are other ways in which RNA helps fine-tune gene regulation in response to various stimuli and environmental factors. For example, when a neuron makes a particular RNA, factors that bind to that RNA can modify how quickly it will be translated into a protein or degraded, or they might dictate the location within the cell to which the RNA will be sent. Through such regulation, it is believed that RNA has the power to induce local changes in a neuron’s connectivity, thereby strengthening or suppressing the responses to particular synapses firing.
Does all this complexity also complicate our understanding of human disease?
Absolutely. For many common disorders, it has been challenging to establish which genes are responsible, and this is particularly true for brain diseases. Take autism spectrum disorders, for example. Initial genetic studies yielded lists of mutations thought to contribute to these conditions, but it turns out that these mutations don’t account for most patients’ symptoms.
One reason for this is that most such genetic research has focused on a subset of patients’ DNA, the approximately two percent of the genome that codes for proteins. For autism, even the best among such studies have failed to identify clinically relevant mutations in two patients out of three. The remaining 98 percent of DNA remains largely unexplored—in fact, some people call it the genome’s “dark matter.”
But we now know that much of this noncoding sequence in fact codes for RNAs. And when you consider just how much influence RNAs have on genes, it starts to look a lot more important.
Newer methods are being developed to sift through entire genomes and predict disease-linked mutations within these noncoding sequences. These are extremely data-heavy studies that require machine-learning algorithms as well as more refined biochemical methods such as CLIP, an approach our lab pioneered. CLIP allows scientists to extract RNA from live brain tissue or from frozen clinical samples and purify the precise points of interactions between those RNAs and the proteins that regulate them.
Recently, we combined CLIP and a similar, DNA-based technology with machine learning to study close to 1,800 families in which one child has autism, and we discovered mutations in the so-called junk DNA that may spur the disease by acting on regulatory RNA or DNA binding proteins. We hope these findings will lay a pathway for how to fill the gaps in our understanding of autism and other complex genetic disorders.
Could the same technology be used with other diseases?
Yes. In mouse studies, we recently discovered that stroke induces a dramatic reduction in the levels of a particular RNA called miR-29. By studying this RNA’s function, we were able to identify a potential drug target for treating a common type of stroke-induced brain damage. We are also applying the method to study memory and fragile X syndrome, and we’re working with other labs to discover noncoding mutations relevant for other brain disorders, viral infections, and different forms of cancer.
We are optimistic that this RNA-centered approach has great potential to further our understanding of all kinds of previously intractable diseases, and potentially come up with new treatments. It’s also promising to be a powerful tool in the laboratory. For example, we will be using it to study the regulation of how synapses connect to neurons with the goal of better understanding both how the brain works normally and how it deteriorates in disease.
Ultimately, mapping sites of RNA regulation and understanding it in greater detail could be a gateway to connecting what we’re learning in the lab to what we’re seeing in the clinic, including how genes and environmental factors contribute to disease. Integrating all this knowledge has been, and will remain, a huge challenge for bioscience. But with the ability to understand and target precise spots of the genome’s dark matter, we might be able to come up with clinically actionable ways to target those spots.