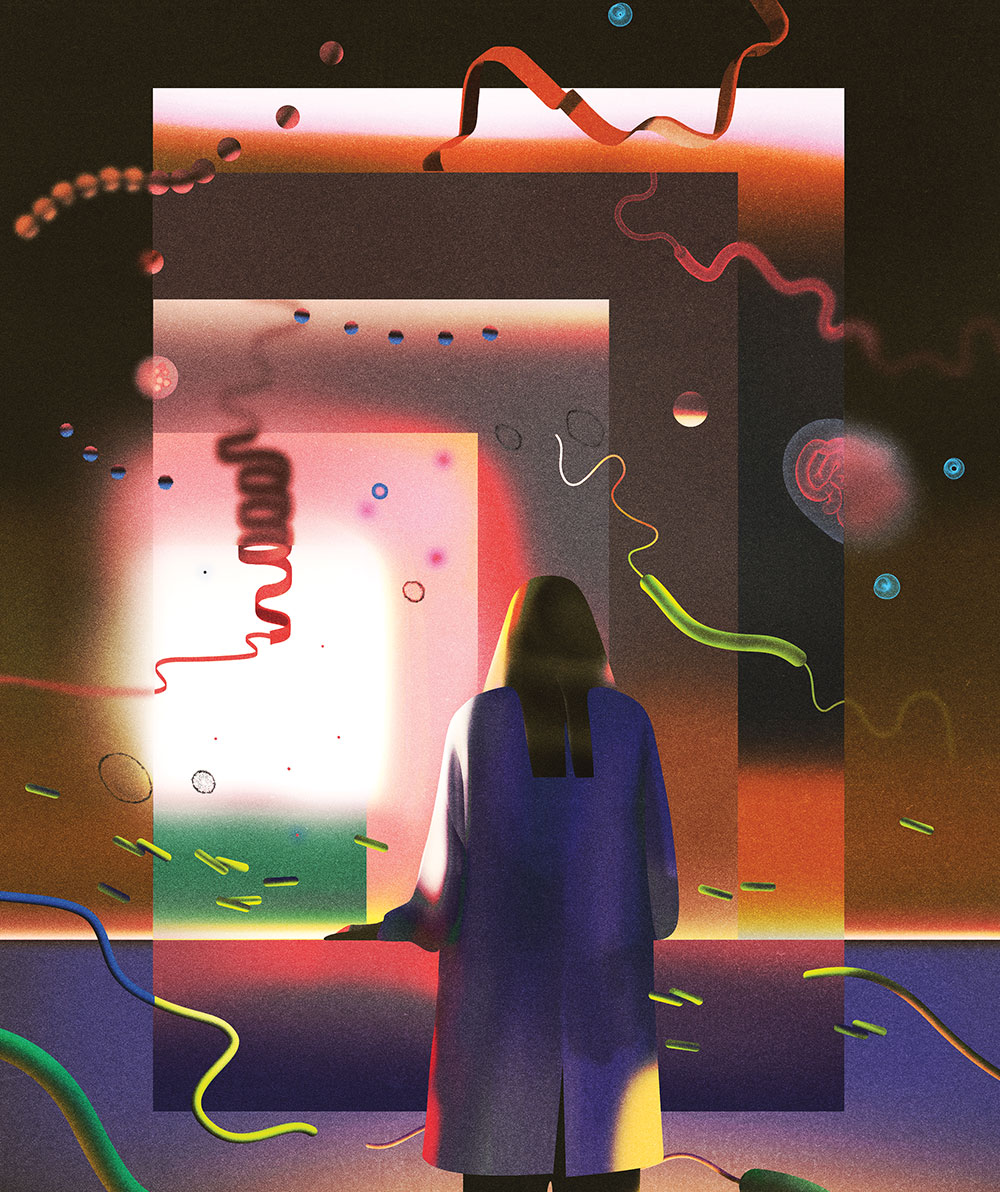
Interview
The shape of things to come
Cryo-electron microscopy is revealing the forms and functions of proteins faster than ever. Jue Chen says it’s already producing revelations that will strengthen medicine and drug design.
By Eva Kiesler & Jen PinkowskiWe tend to think of machines as being of human origin—objects made by us and for us and that wouldn’t exist without us. But proteins mastered molecular machinery billions of years ago, long before the first tetrapod crawled onto land. In short order, protein machines began to operate all of life. We can talk, run, communicate, sleep, and think because proteins are doing all the heavy lifting and smooth automation necessary for cell function: pumping, transporting, linking, bonding, grasping, shunting, filtering, and disposing of materials.
But much about how proteins operate is still mysterious. As with any cleverly designed device, their function follows their form, and any given protein can take on several forms, or conformations. So with every new conformation that the protein folds itself into comes a new function. To truly understand what it’s up to, you have to see it in action.
Take the maltose transporter protein complex, whose job it is to absorb sugar molecules into bacterial cells. It’s been scrutinized for decades by structural biologists who hoped it would serve as a skeleton key to unlock the mysteries of all ABC transporters, molecular pumps that move cargo such as sugar molecules into or out of the cell. Its components had all been identified, but how they worked together as a functional unit eluded everyone—until Jue Chen, a specialist in ABC transporters, captured the first snapshots of the full maltose transporter in action using X-ray crystallography, a method in which molecules are painstakingly purified and crystallized and then studied with radiation.
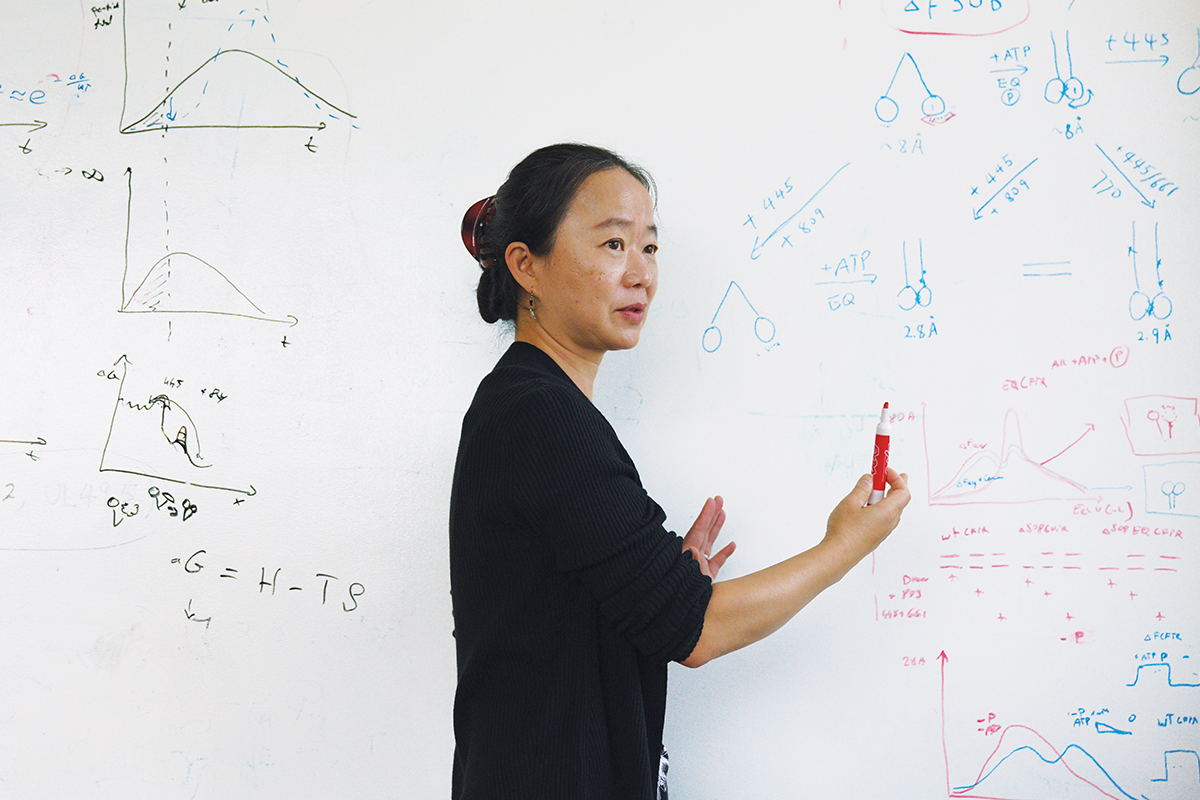
It was an utterly revelatory moment for her. “It was beautiful; I could see every atom,” says Chen, the William E. Ford Professor and head of Rockefeller’s Laboratory of Membrane Biology and Biophysics. “It’s incredible when a new structure gives you that intuitive understanding of how a process works. It’s the only way to understand how biology happens at its most basic level.”
Historically, such revelations about molecular architecture and dynamics have been hard won, with many scientists spending years or even a lifetime to solve the structure of a single protein. Many molecular components are too fragile for X-ray crystallography’s dehydration process and simply collapse into chaos. But in the past decade, breakthroughs have been coming faster than ever for structural biologists like Chen thanks to a new technique called cryo-electron microscopy (cryo-EM). Insights that used to take years to emerge can now appear in months.
Not surprisingly, Chen’s research has bloomed too. With new ways to watch molecular events up close, her lab is making swift progress in exploring ABC transporters, generating findings that might one day be used to prevent cancer cells from spitting out killer drugs or to treat diseases such as cystic fibrosis.
We asked Chen to tell us more about what this methodological revolution means for medicine and science, and what other advances we can anticipate.
What was it like to be a structural biologist when you began your career?
The work was very different from what it is today. To solve the structure of a protein, we had to purify it and make it form a crystal that we then probed with X-ray radiation. And while a simple compound like table salt will easily arrange itself into a neat lattice, biomolecules tend to be flexible and fragile, making the crystallization process incredibly demanding and time-consuming. To succeed, you had to be a very good biochemist—and you had to be extremely patient and careful.
I’ve spent years in the lab trying and failing to grow crystals from the molecules I wanted to study. We used high-throughput technology and tried thousands of conditions at a time. We tried different chemicals. We tried modifying the protein to hit the right spot. It could take many, many years and thousands and thousands of trials.
There have been many proteins I was very interested in that never crystallized. This was the case with CFTR, for example, a protein that moves chloride ions across the cell membrane and whose mutation is responsible for cystic fibrosis, a devastating lung disease. One piece of the protein responsible for regulating the channel is so floppy that crystallization is virtually impossible. I was never able to make it work.
That sounds frustrating. How did cryo-EM impact your research?
Cryo-EM solved our problems. When I joined Rockefeller in 2014, a team at the University of California, San Francisco had just published a landmark paper on the use of this technique for structural biology. Rockefeller immediately allocated funds to set up the instrumentation, and several of my faculty colleagues and I raced to learn the new technique ourselves. With cryo-EM, we no longer needed to grow crystals; instead we could suspend the molecule of interest in a buffer solution and flash-freeze it. This technique not only enables us to solve structures that were previously beyond reach but also makes it possible to simultaneously capture many snapshots of a molecule as it transitions from one state to the next, giving us unprecedented insight into its dynamics.
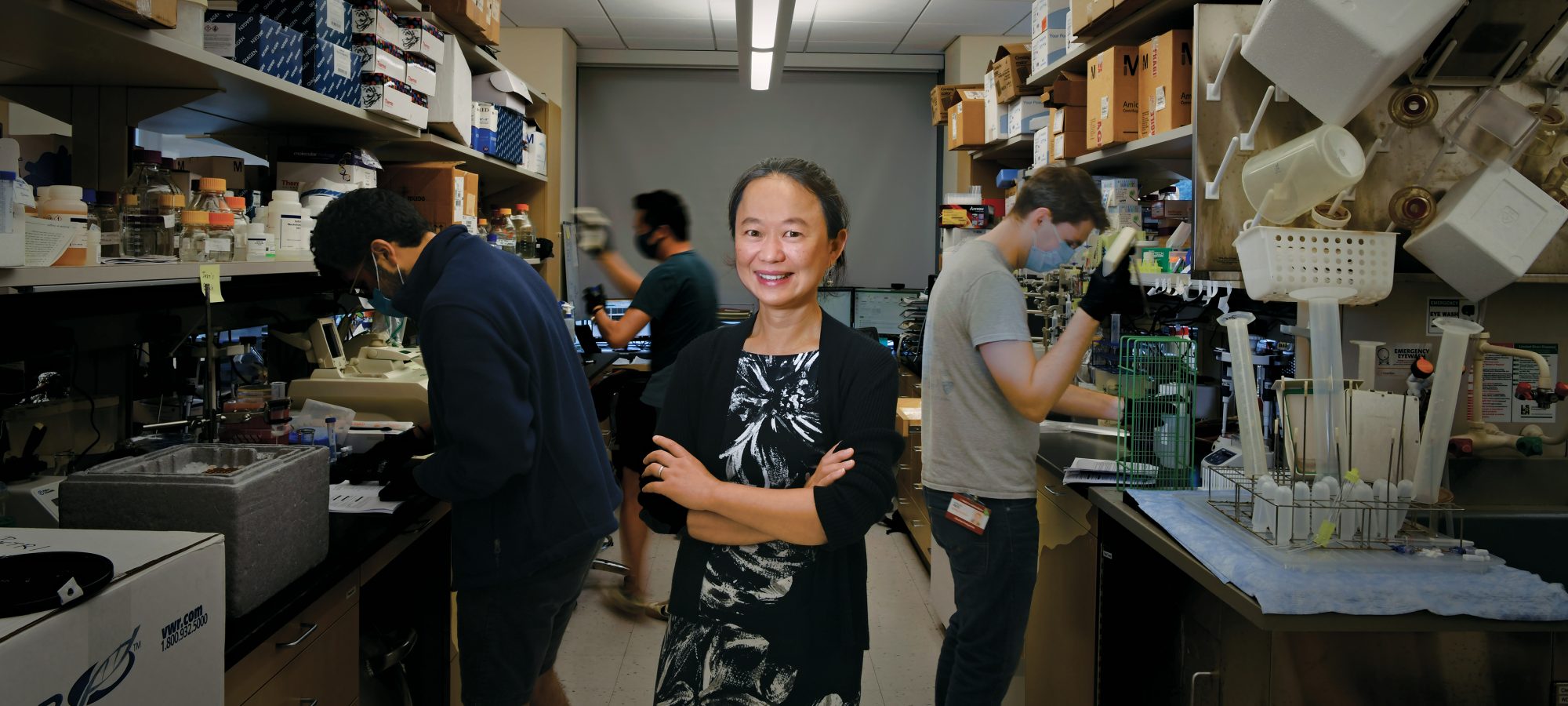
You basically take many 2D pictures of the purified molecule, and then you reconstruct its shape in 3D. With every step, the molecule is telling you, “This is what I look like.” We also stitch together snapshots of the same machinery at different stages of its work and put them together as a movie.
We were just so excited because with all these projects we worked on for years, we couldn’t get a hint of crystallization. Then boom, boom, boom—one after another, we solved them by cryo-EM. It felt so empowering.
How might this new knowledge improve medical treatments?
It’s already beginning to enable new approaches like rational drug design—the idea of synthesizing drugs based on what we know about disease-related molecules and the way they bind with their molecular partners, or ligands. Now that it only takes a few months to produce structural renderings, we can look at a pair of interacting molecules and ask, “What will it take to make their binding more efficient? What if we add a certain chemical group at this or that location?” It means we will be able to iteratively optimize drugs to eliminate side effects, for example, or to improve their uptake in cells and tissues.
It may soon become possible to optimize drugs to eliminate side effects or improve uptake in cells and tissues.
I’m very excited about computational “docking” techniques that make it possible to discover new ligands. Until now, most existing drugs have been identified by brute-force searches in which millions of molecules had to be tested. Scientists are developing increasingly smart software that can analyze the properties of molecules and predict the ones with the highest chance of forming a stable complex with a target—a mark of the molecule’s potential as a future drug.
Another big opportunity for drug discovery is to study the function of existing medications. We don’t actually know how many of our best drugs work, and learning how one medication does its job might open the door to developing new ones based on the same principles.
Could you give us an example?
My lab recently solved the mystery of how CFTR correctors, a class of cystic fibrosis drugs, work. It turns out that the CFTR protein has an internal cavity that makes it intrinsically unstable. This defect is exacerbated in people with various kinds of mutations, preventing it from folding properly. As a result, it gets prematurely degraded inside the cell. We were able to understand how CFTR correctors fix this problem: They bind inside the cavity and fill it, stabilizing CFTR and prolonging its life span (read more about the lab’s work on CFTR correctors in “How misfolded proteins get into shape”).
No other drug has previously been shown to act in this way, and we suspect the same mechanism could be employed to design new drugs against a range of diseases. Beyond cystic fibrosis, there are hundreds of illnesses believed to be caused by the inability to produce a correctly shaped protein, including neurodegenerative conditions like Parkinson’s and Huntington’s. Could these diseases also be treated by correcting a misfolded protein, and does that protein have internal cavities like CFTRs do? We are doing the experiments to find out.
Tell us more about how computational tools are advancing the field. What about AI, for example?
AI tools are making it possible to predict the structure of a protein from its amino acid sequence. Scientists have tried to make such predictions for decades based on the spatial configurations a particular stretch of amino acids will have due to its chemical properties. The new AI tools perform much better. They use machine-learning algorithms to sift through a database and pick the shape most likely to match a sequence.
But AI predictions are not always accurate, and they will never replace experimental techniques. Still, in some cases they can save a lot of time. People in the field increasingly rely on them to guide experiment design, for example.
So, what might be the next revolution?
Thus far, we’ve mainly been looking at proteins in isolation. We break the cell open, take the protein out, and work with it in vitro. But many proteins don’t fold or function properly outside of their natural environment, so there’s always a risk of collecting bad data.
In the future, emerging technologies will enable us to look at molecular structures in living cells—maybe even within living tissues. For example, we might soon be able to use cryo-EM to determine a protein’s cellular distribution and watch it interact with other proteins in its surroundings, all while still being able to see its structure with near-atomic resolution. It will be amazing.
Do you ever get nostalgic for the old days of crystallography?
Sure—determining structures was very challenging back then, and I love a challenge. It’s a very special feeling when you get to see something nobody has seen before, even more so if you’ve been working on it for years.
Still, it’s hard to ignore that cryo-EM has made our day-to-day work more interesting and versatile. It has freed up so much of our time, giving us the opportunity to ask more complex questions and do all kinds of biological experiments. Everyone in my lab has a structural biology component, but they do many other things as well. Now we are looking for molecules that regulate the function of a protein, making it more active or inhibiting its function. To be honest, our field is more fun now than it’s ever been!