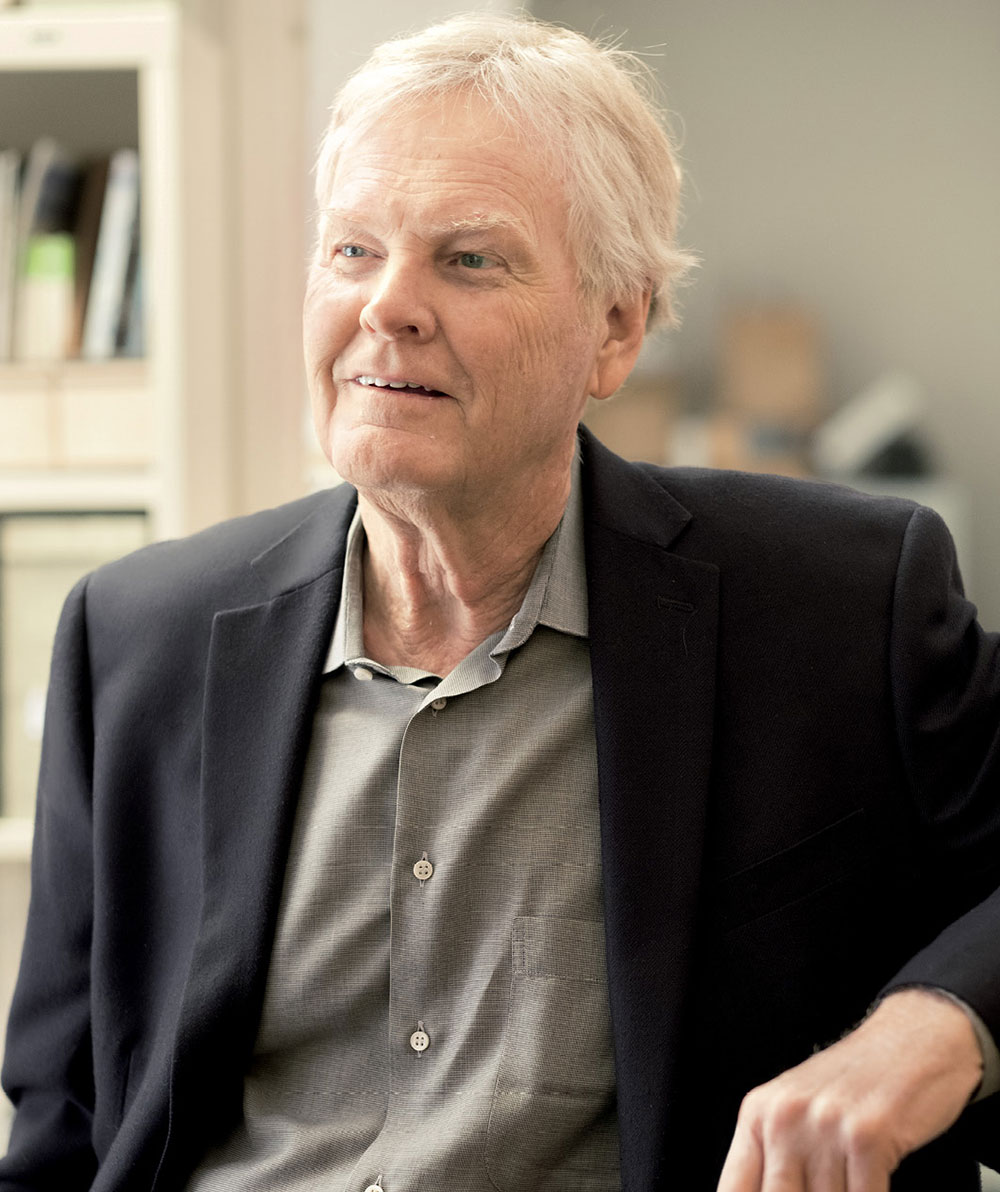
Interview
Michael W. Young
Tracking time isn’t something we do with just our brains and our wrists. Most cells in the human body can mark the passage of Earth’s 24-hour rotation. Meet the scientist devoted to the biology of the day.
By Zachary VeilleuxIt’s easy to tell if a fruit fly is awake or asleep. If it’s flying around, it’s awake. If not, it’s dreaming of spoiled mango.
When Michael W. Young began working on circadian rhythms in the early 1980s, he needed some way to automatically record his flies’ sleep-wake cycles. Rockefeller’s in-house machine shop built him a custom tool: a breadbox-sized piece of equipment capable of monitoring five flies at a time, causing a signal every time one of its occupants ran past a sensor. Active flies would zip back and forth endlessly; those that didn’t trigger the device were asleep.
It was a simple concept, but it proved indispensable. Over the years, Young and his lab members put thousands of genetically altered flies in the machine’s tiny glass tubes (and those of its successors), and the results led to discoveries of the key genes and proteins all organisms use to regulate their internal clocks—discoveries for which Young received the Nobel Prize in Physiology or Medicine this year.
We spoke to Young, who is the Richard and Jeanne Fisher Professor, about his favorite biological mechanism a few days after the prize was announced.
Why did you decide to study clock genes?
I didn’t at first. Originally I was a Drosophila geneticist, looking more generally at how genes of animals might differ from those of bacteria. I was also interested in transposable elements—segments of DNA that jump from one location on the genome to another—and trying to identify what role they had in the life of a fly. On the way I became interested in a gene, called period, that was related to circadian rhythms.
I had first come across the period mutations as a graduate student, and thought it was something I might like to work on. The more I looked at it, the more it revealed itself to be a very pretty piece of biology. It had a lot of mystery, and the ramifications turned out to be far beyond what we imagined.
So you narrowed your focus?
We dropped the other work and tried to isolate the period gene. That’s when we built the sleep-wake recorder, so the postdocs wouldn’t have to stay up all night with a stopwatch, watching flies walk and sleep. We used it on mutant flies that were arrhythmic—they had no regularity to their sleep patterns—and inserted DNA into them that we thought contained the period gene. Every morning we’d come in and read the printouts logging their sleep patterns. In those experiments we were able to restore rhythms to arrhythmic flies, proving we had our gene.
There was a rule of thumb in genetics at the time that if you can’t find what you’re looking for in the first 200 strains, it’s not there. And we’d done that. So we decided to go past 200, and we in fact had to search 7,000.
“There was a rule of thumb that if you can’t find what you’re looking for in the first 200 strains, it’s not there. We in fact had to search 7,000.”
An important early finding was when we found a translocation in the genome. We saw that a snippet of DNA breaks from the tip of the X chromosome and integrates itself into the fourth chromosome. Flies with this translocation would have no discernable pattern of sleep. This was our earliest indication of where exactly the gene was in the chromosome we were studying, and we eventually found that this translocation was breaking a non-coding part of the period gene—meaning this was a problem not of the gene itself, but of its regulation. That was a big clue.
What’s the most important thing you’ve learned about how these cycles work?
This is a story not of a single big discovery, but of the accumulation of small discoveries over many years that eventually led to a fairly clear picture of how cells track time.
Besides the period gene, we found several other genes involved in this system. Eventually we discovered that period works with a gene called timeless. The protein products of these two genes accumulate together and pair up in the cell’s cytoplasm. When a certain threshold is reached, they migrate into the nucleus where their presence stops further production of the two proteins by shutting down the corresponding genes.
It then takes a while until the proteins clear out and the brake on their genes is released—at which point a new cycle begins. This cycle of accumulation—driven by a few other genes that we have identified more recently—generates a consistent delay and sets up a daily rhythm.
Finding timeless was the key, but it wasn’t easy. There was a rule of thumb in genetics at the time that if you can’t find what you’re looking for in the first 200 strains, it’s not there. And we’d done that. So we decided to go past 200, and we in fact had to search 7,000. We decided the 200 rule was an absurdity.
Why do all our cells need to know what time it is? Why isn’t that something that the brain simply keeps track of?
So much of our biology depends on what time it is, and having seamless coordination between different tissues is critical. This means each organ needs to do its job at the right time without having to wait for another organ, like the brain, to tell it what to do.
For example, firing up the body from a sleeping state to one where we can function requires time-dependent regulation across different tissues. Things like blood pressure, growth hormone, cortisol, heart function, muscle strength—all are changing over the course of the day. Some are changing more in anticipation of the cycle of daylight and temperature, but others are cued to food. This allows for critical body functions to be reproducible, and it gives you the ability to be ready for recurring daily events—like meals—at the right time.
What happens when we colonize another planet where the days are longer or shorter than 24 hours? Will we adapt?
Initially we won’t. We’ll live in perpetual jet lag, or else we’ll create an artificial environment to mimic the rhythm we are used to. But eventually, as a species, we will evolve. We have faced this problem before: the days were shorter hundreds of millions of years ago, when Earth rotated at a higher rate.
One of the things our research has shown is just how easy it is to manipulate these clocks. By changing just a couple of letters in the DNA code, we can create a fly with a four-hour change in periodicity. We’ve made flies with clocks ranging from 16- to 40-hour cycles that run just as well as the regular, 24-hour clock. When the time comes, evolution will be ready.
One could imagine a pill that would allow people to function on less sleep, to move to a 40-hour cycle so they could be more productive. Is that realistic?
The problem is you would need to move the clocks running in each of your tissues all in the same direction and at the same rate. And it’s hard to devise a single medication that can accomplish that. If they get out of sync it’s like jet lag: you might be able to adjust your sleep schedule relatively quickly, but you’ll still get hungry at the wrong time. These timekeeping systems can have a lot of inertia, and some organs require more reinforcement than others before they adjust.
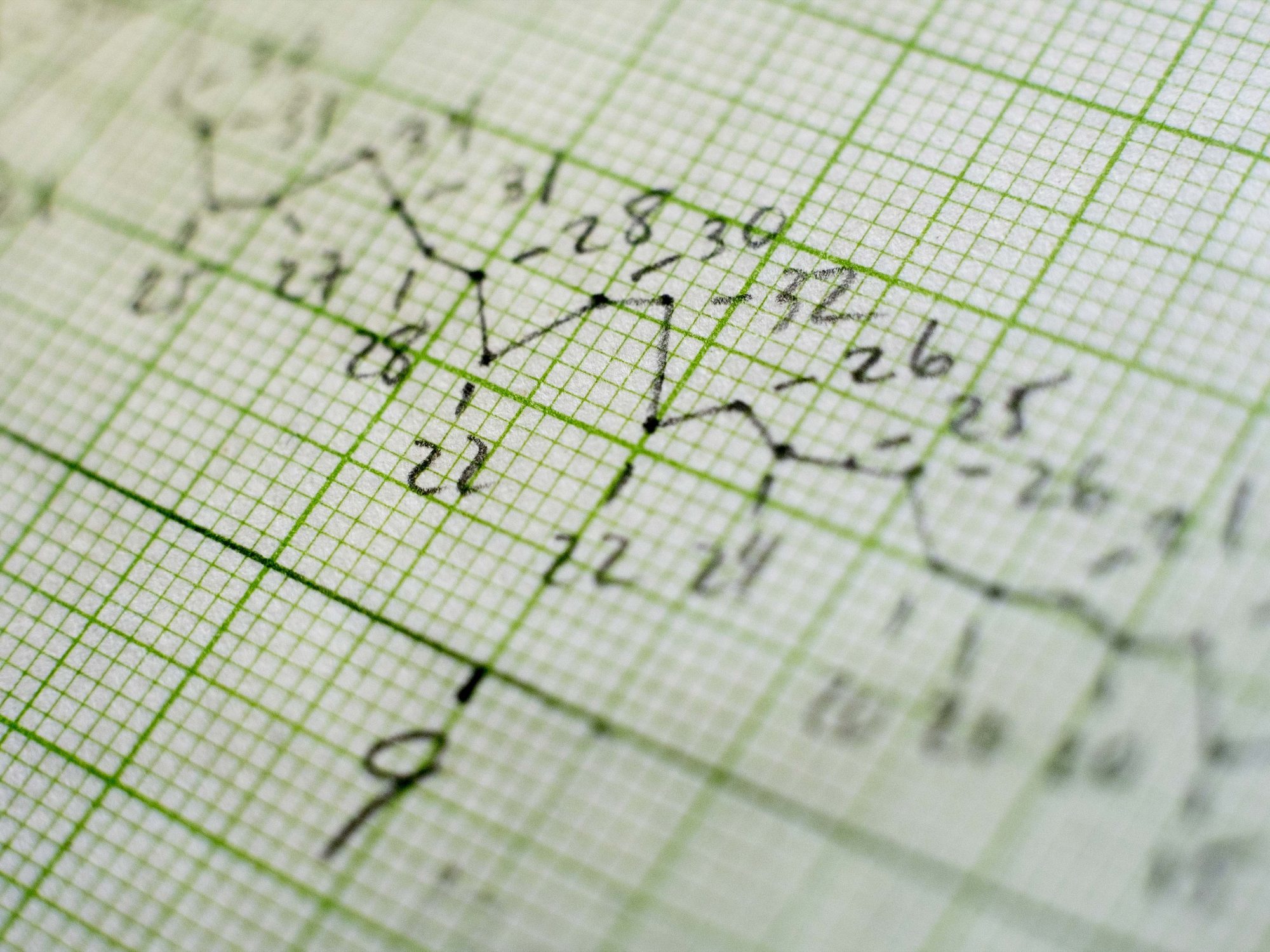
What about devising a cycle-restoring medication to help people with sleep disorders?
There is some potential here. One thing we’ve found, looking at people who have a syndrome called delayed sleep phase disorder, in which bedtimes are consistently shifted two to three hours, is a common mutation that produces a hyperactive protein and is present in the clock of every cell. If you create a medicine that interacts specifically with the version of the protein produced by this gene, you could eliminate it from the system. Every cell would lose the dominant influence of that variant and presumably be restored to normal function.
But in general, we see a lot of genetic variability among people with sleep problems. In many cases, the ability to have an effective therapy will be very much dependent on whether there is a genetic change and, if so, which mutation you have.
Has what you’ve learned in fruit flies translated nicely into people?
Much more so than I would ever have thought at the outset of this work. Fly clocks and human clocks, it turns out, mostly run on the same genes and proteins, and even when there’s a variation it typically doesn’t change the way the whole system works.
For the past several years we’ve actually been doing clinical work to study the effect of various gene mutations in volunteers. Thanks to vast databases I can sit at a computer and look at every gene I know contributes to how these clocks run. I can see what kinds of mutations have been found, and I can make initial guesses about what might cause shifts in sleep-wake cycles.
We can then test those mutations in cellular systems and ask how frequently they are seen in the population. If it’s frequent, we have a good chance of being able to find people who will volunteer for our studies—and we have an idea what to look for.
How will the Nobel Prize affect work in this field?
My hope is that people will begin to talk about circadian rhythms with a level of understanding they already have for things like DNA. A Nobel Prize can draw attention to this field, in which so much growth has occurred in recent years, and translate it to something that everybody thinks they should know something about. This work is not hard to grasp, and the implications are very broad.
Circadian rhythms are a major component of our biology—we are rhythmic organisms.