Feature
A transformative moment for RNA
Once considered DNA’s rote protein generator, RNA has fully emerged from the shadow of its more famous cousin. As researchers discovered its myriad types—small, long, noncoding, small interfering, piwi-interacting, micro, ribosomal, small nucleolar, and many others—they’ve also revealed how central RNA is to a range of biochemical tasks, from regulating gene expression to mobilizing other molecules into action. Far from a mere go-between for DNA and ribosomes, RNA actually possesses a diversity of form and versatility of function that is virtually unrivaled in biology.
By Joshua A. Krisch and Jen PinkowskiFrom the outset, Rockefeller scientists have played a crucial role in transforming our understanding of RNA. Their foundational discoveries illustrate how cells use it to respond to environmental signals; pinpoint how its dysregulation leads to disease; underpin RNA-based therapeutics for formerly untreatable conditions; explicate its role in the quick-change evolution antibiotic-resistant bacteria are capable of; and help exploit RNA’s complex architecture for drug potential, among other breakthroughs. Together, their work is demonstrating how RNA’s impressive skills can be harnessed to fine-tune human health.
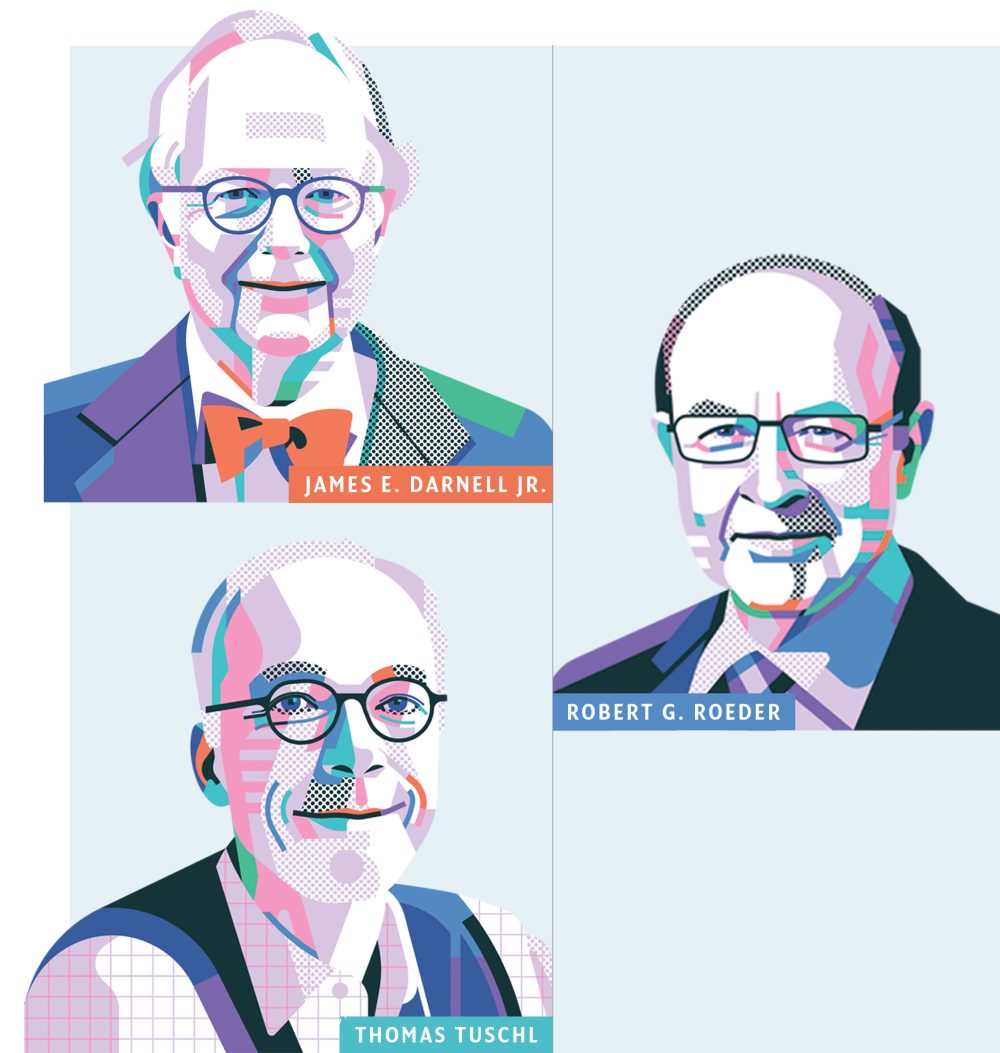
James E. Darnell Jr.
When James Darnell graduated from medical school in the mid-1950s, no one knew how profoundly the science of RNA would revolutionize vaccine development and disease treatment—and even alter our understanding of heredity itself. But already the burgeoning field was raising huge questions, and Darnell saw a challenge.
Only a decade had passed since scientists had demonstrated that DNA was the principal vehicle of heredity, and none other than Francis Crick had just proposed that another nucleic acid was required to turn the information it contained into proteins. Researchers were racing to discover this missing link in protein synthesis in hopes of filling out the story of how genetic information is passed on.
“I knew what my future was—to find this messenger RNA in our cells,” Darnell says. While working at the National Institutes of Health, Darnell helped develop a technique for extracting RNA from viruses to better understand how they hijack cells and force them to produce viral proteins. Less than a decade later, he used a version of this technique to investigate mRNA in animals, solidifying our understanding of RNA’s central role in carrying genetic information and directing protein synthesis. “No one had ever successfully removed all of the RNA from an animal cell intact,” Darnell says. “But I knew that I could do it.”
Over his next five decades at Rockefeller, Darnell, today the Vincent Astor Professor Emeritus, conducted work on RNA and the expression of genes within the cell nucleus, a.k.a. nuclear transcription. This research built the backbone of modern molecular biology.
“No one had ever successfully removed all of the RNA from an animal cell intact. But I knew that I could do it.”
The Darnell lab focused on understanding how signals received at the cell surface affect nuclear transcription, in part by studying the proteins that relay those signals and initiate the process. By clarifying how these proteins affect gene activation, Darnell and his colleagues proved that they play a key role in regulating gene expression—and in controlling cell growth and immune responses, both of which become dysregulated in cancer. Darnell ultimately confirmed that aberrant activation of these proteins could lead to uncontrolled cell growth, a finding that spurred the development of novel drugs that are now in clinical trials for cancers such as leukemia.
These foundational insights led researchers to uncover the pivotal surface-to-nucleus “Jak-STAT” pathway—partially named for the STATs (or relay proteins) he first identified—which underlies numerous processes from inflammation to cell specialization and since has been implicated in disorders as varied as susceptibility to fungal infections and dwarfism.
Through his previous ingenious detection of the long RNAs that serve as precursors to mRNA, Darnell had paved the way for discovery of RNA splicing, filling a critical gap in our understanding of gene expression and providing the first full explanation of how cells translate genes. By explicating surface-to-nucleus signaling, Darnell showed how cells can use RNA to quickly respond to changing environmental signals.
The revolution was now well underway. –JK
Robert G. Roeder
As a boy, Robert Roeder enjoyed taking apart clocks and putting them back together to see how they worked. As a scientist, he became enamored of the idea of doing the same thing with cells.
Specifically, Roeder wanted to unpack the mechanisms that drive transcription in animal cells—in particular, RNA polymerase (RNAP), an enzyme that orchestrates the intricate process of gene activation and repression by binding and unwinding DNA.
“I was fascinated by how RNAP initiates the decoding of each cell’s DNA blueprint,” he says. “Understanding how and when the cell reads DNA—I couldn’t think of anything more essential to study.”
While still a graduate student in the late 1960s, Roeder discovered that transcription in animal cells involves not just one RNAP but three, contributing fundamentally new insights into gene expression and paving the way for subsequent research into gene regulation and heredity itself. Building upon this work, Roeder was the first to elucidate the general functions and complex structures of the RNAP trio, known as RNAPs I, II, and III. He ultimately found that each of these three enzymes performs a distinct function in the synthesis of three major classes of RNA that are collectively necessary for protein production: RNAP I is central to ribosomal RNA, RNAP II to messenger RNA, and RNAP III to transfer RNA. He also discovered the first of some 1,600 gene-specific transcriptional activators responsible for regulating cell-specific gene expression programs in animal cells.
“Understanding how and when the cell reads DNA—I couldn’t think of anything more essential to study.”
At Rockefeller, Roeder, the head of the Laboratory of Biochemistry and Molecular Biology, determined that, in eukaryotes, each of these enzymes requires a distinct set of general accessory factors to kick off transcription, quite unlike their close cousins in bacteria. Notably, however, these general bits of cellular machinery are used differently by different cells, leading Roeder, who is also the Arnold and Mabel Beckman Professor, to suspect that RNAPs and accessory factors alone weren’t enough. Deploying RNAPs for specific needs, he realized, must require not only gene-specific transcriptional activators but also a set of specialized coactivators.
He found this to be especially true of RNAP II, and he has applied this pivotal insight to the study of numerous biological processes. His work on fat cell development and differentiation, for instance, has shown how precise regulation of RNAP II is essential for maintaining metabolic balance and energy storage; he is also exploring how factors regulating RNAP II contribute to the process by which the body burns fat to generate heat. Both results offer new ways to think about managing obesity and metabolic disorders.
Roeder’s research has also revealed that disruptions in RNAP II regulation may lie at the heart of immune disorders and blood cancers. “Understanding RNAP regulation is a powerful tool for addressing disease,” Roeder says. –JK
Thomas Tuschl
When Thomas Tuschl began his career in the 1990s, the study of RNA was at a turning point.
Scientists were beginning to understand that RNA does more than just carry genetic instructions from DNA—it can also trigger and control reactions within cells. This discovery opened up new possibilities for using RNA in biotechnology and medicine. And one class of RNA, known as small RNA, showed unique potential. With it, scientists could start imagining a future in which precise RNA-based therapies lead to treatments for genetic disorders, cancer, and infections.
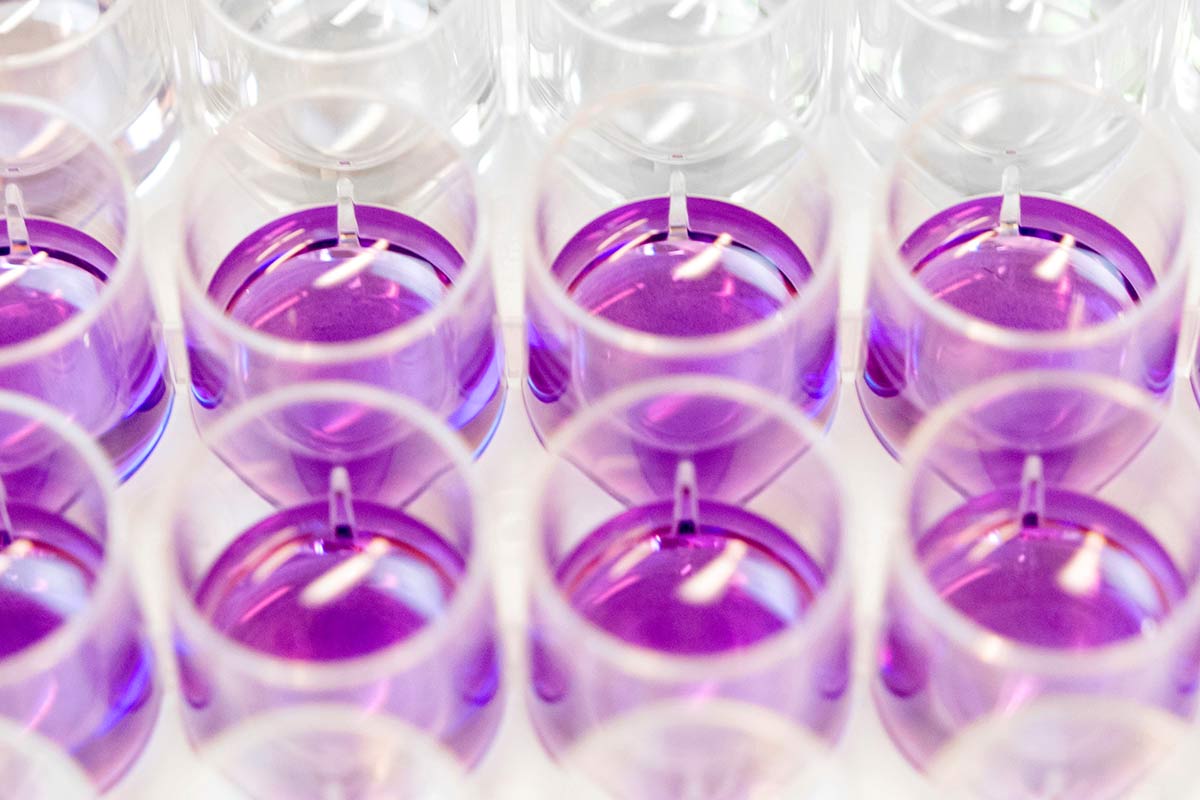
Of the many classes of genome-encoded RNAs, only small RNA is involved in turning genes on and off. Tuschl, who heads the Laboratory of RNA Molecular Biology, was instrumental in explaining how these regulatory RNAs work, and his insights have opened the door to RNA-based therapeutics for once-untreatable diseases by targeting the mechanisms that control gene expression.
Early on, for example, Tuschl proved that RNA interference (RNAi), the process by which small RNAs suppress gene expression, operates in mammalian cells, making years of laboratory research on other organisms suddenly relevant to humans. But his pioneering achievements haven’t stopped there.
“Small RNAs play a complex role in maintaining genomic integrity and regulating gene expression.”
Tuschl was also the first to uncover the critical role of piwi-interacting RNAs (piRNAs) in preserving genome stability within the germ cells that give rise to sperm and eggs. He found that this type of small RNA acts as a kind of genomic guardian, silencing disruptive elements to ensure healthy germ cell development and fertility. His work on microRNAs (miRNAs), meanwhile, revealed those molecules to be the master regulators of gene expression, binding to and destabilizing specific mRNA sequences to fine-tune gene activity.
Tuschl has channeled his numerous RNA-related discoveries into practical and life-saving applications. In addition to demonstrating that miRNA plays a central role in cancer, he has founded two pharmaceutical companies dedicated to developing drugs that modulate RNA to combat a range of genetic and autoimmune disorders, including lupus and Parkinson’s disease.
“Small RNAs play a complex role in maintaining genomic integrity and regulating gene expression,” says Tuschl, who is the F. M. Al Akl, M.D. and Margaret Al Akl Professor. “As a result, understanding them is crucial to developing certain new therapeutic strategies.” –JK
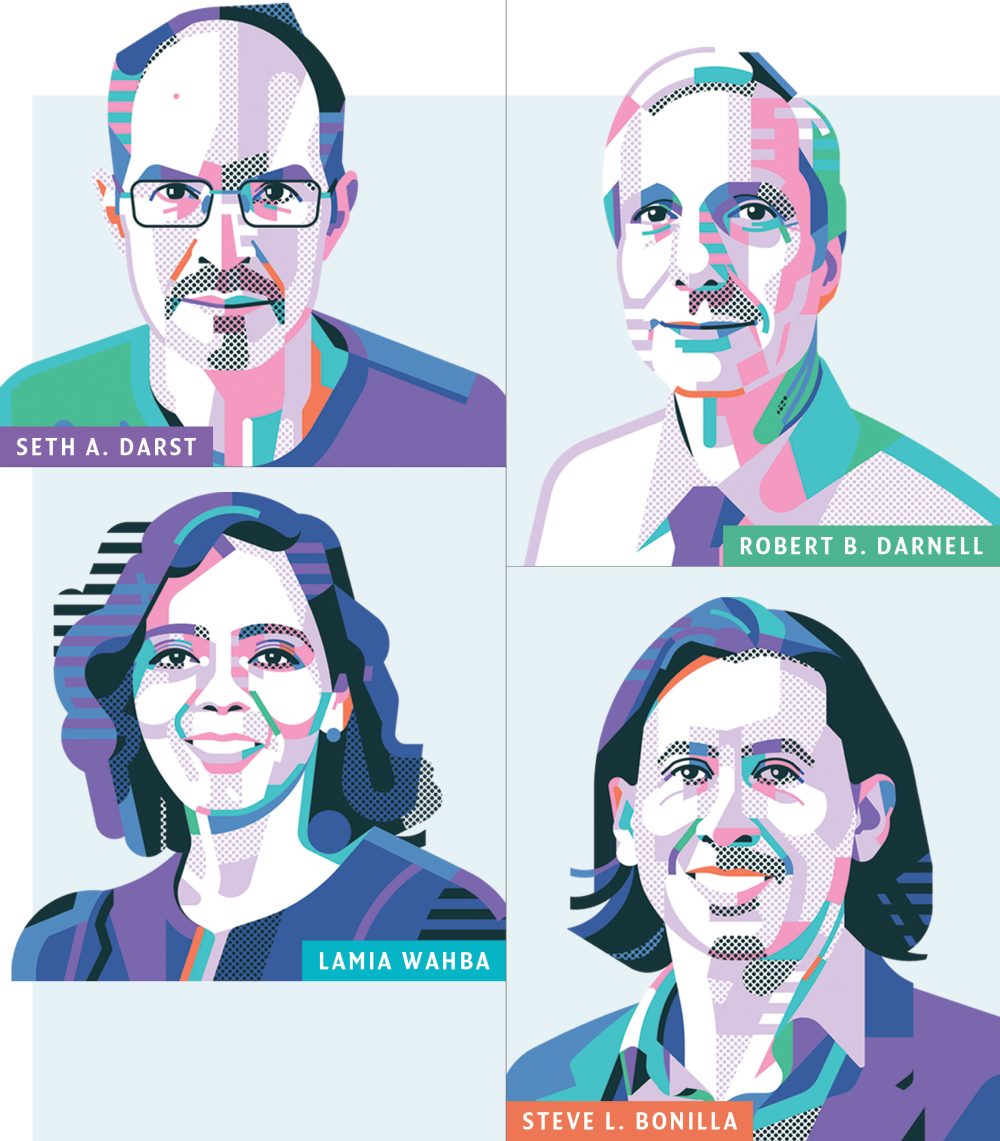
Robert B. Darnell
Robert Darnell never intended to join the family business.
Darnell’s father and fellow Rockefeller researcher, James, helped launch the field; Robert had no intention of following in his footsteps. “When I got into science, the only thing I didn’t want to work on was RNA,” he says.
But when the younger Darnell dove into the study of neurodegeneration—presumably a safe distance from his father’s legacy—“lo and behold, there it was.” Through sheer serendipity, he discovered the proteins that regulate RNA metabolism in brain cells, illuminating how RNA helps drive brain function.
Prior to Darnell’s discovery, whatever suspicions molecular biologists may have harbored about the role of RNA in the brain, technological limitations prevented serious study of the subject: No method existed to explore RNA and its binding proteins in detail.
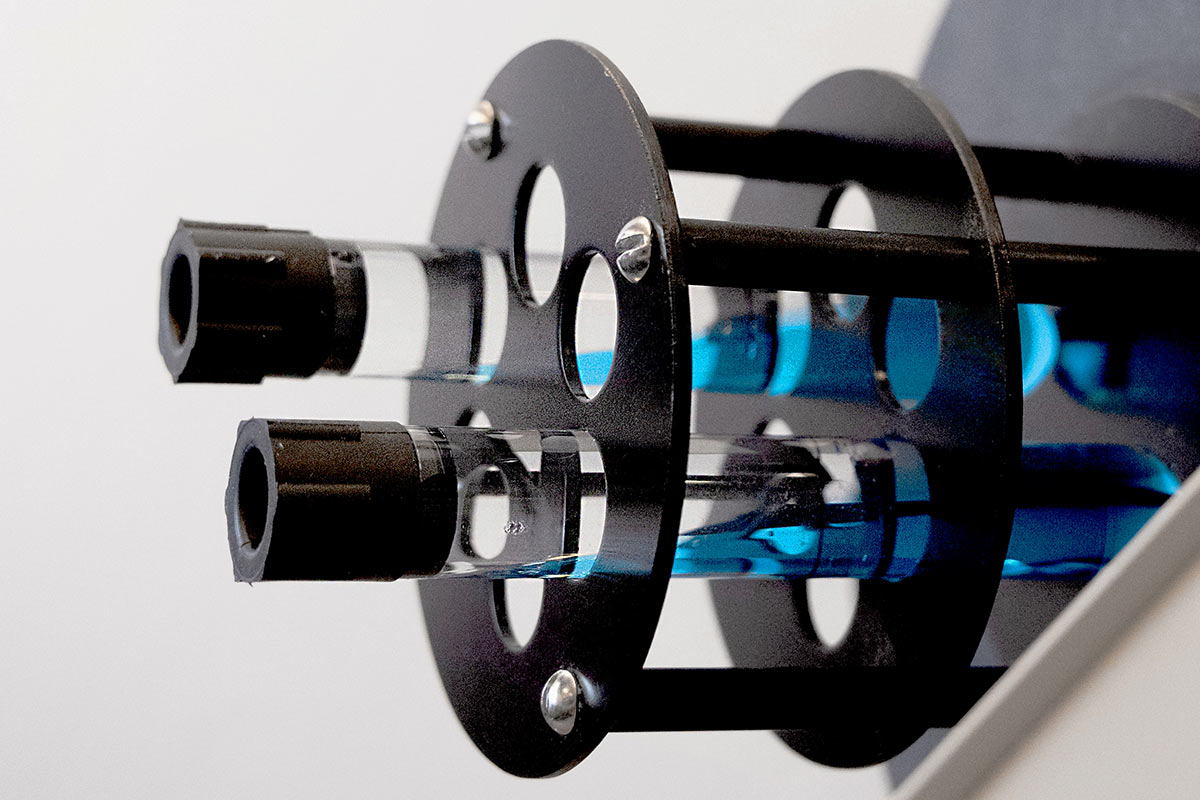
Darnell changed all that. His groundbreaking CLIP technology allowed him to produce detailed, high-resolution maps of where specific proteins attached themselves to RNA molecules, revealing the intricate regulatory networks that control gene expression.
That work markedly advanced the study of how RNA and RNA-binding proteins contribute to brain cell function—and, in the case of neurological disorders, dysfunction. Darnell, who is the Robert and Harriet Heilbrunn Professor and head of the Laboratory of Molecular Neuro-oncology, ultimately discovered that disorders such as autism, epilepsy, and neurodegenerative diseases all have strong connections to these proteins, revealing how disruptions in RNA regulation can lead to significant changes in the brain and establishing a whole new paradigm for understanding and addressing neurological conditions.
“There’s a lot of hype around RNA. And I’m on the high end of the hype.”
Darnell’s work on RNA and its associated proteins not only deepened our understanding of disease but also suggested entirely new treatments. For example, the insights that Darnell and his colleagues have gleaned into how RNA-binding proteins regulate neuronal gene expression and stress responses are paving the way for innovative therapies designed to slow or even halt the progress of Parkinson’s disease. And their investigations into the role that RNA-binding proteins play in regulating immune responses have led to new therapeutic targets for rheumatoid arthritis.
It’s an RNA-heavy docket for someone who once balked at studying the molecule at all. But Darnell has no qualms about where he wound up.
“There’s a lot of hype around RNA,” Darnell says. “And I’m on the high end of the hype.” –JK
Seth A. Darst
Every cell on earth transforms its genetic code into marching orders through a precise three-step transcription cycle. Key to this process is RNA polymerase (RNAP), a hefty enzyme that builds RNA molecules from strands of DNA. Stopping RNAP in its tracks is a surefire way to interrupt the cycle; several antibacterials and antivirals work in just this way.
And yet well into the 1990s, the interior makeup of the RNAP used by bacteria remained a mystery. When viewed through a transmission electron microscope, it looked so much like a blurry smear that Seth Darst and his fellow structural biologists called their work “blobology.” They eventually produced mildly sharper images they called Pac-Mans—“a cool nickname, but hardly informative in terms of molecular mechanisms,” says Darst. But if they wanted to find fresh targets for new antibiotics—desperately needed in the face of growing antimicrobial resistance—they needed to reveal its inner structures.
“Cryo-EM is revealing processes of the transcription cycle we’ve not yet seen, on timescales we’ve never achieved before.
In 1999, Darst, Rockefeller’s Jack Fishman Professor and head of the Laboratory of Molecular Biophysics, captured the first high-resolution images of a bacterial RNAP structure. Since then, he’s pursued a complete understanding of RNAP’s structures, biochemical interactions, and regulators as it performs its role in the transcription cycle. Despite cryo-EM making their view of RNAP exponentially sharper than in the days of blobs and Pac-Mans, “there’s so much we still don’t understand about the chemical steps RNAP goes through as it adds one nucleotide at a time to an RNA chain. We also don’t understand the likely hundreds of transcription factors that regulate the cycle.”
His lab’s insights into polymerase structures have revealed how rifampicin, a key component of tuberculosis therapy, works. During the COVID pandemic, his group, working with Elizabeth Campbell, who now heads the Laboratory of Molecular Pathogenesis as the Corinne P. Greenberg Women & Science Professor, illuminated unknown characteristics of SARS-CoV-2 by creating an atomic-level resolution view of its replication system, and provided key evidence explaining how the antiviral remdesivir can work. Darst continues to sharpen the view of RNAP’s inner workings. His lab is collaborating with researchers at Rockefeller and at the New York Structural Biology Center to build the next generation of tech: a time-resolved cryo-EM machine that takes RNA polymerase and DNA, mixes them together on a cryo-EM grid, and then freezes the stew in just 100 milliseconds.
“That can show us processes of the transcription cycle that we’ve never seen, on timescales we’ve never achieved before,” he says. –JP
Steve L. Bonilla
RNA molecules are commonly depicted as squiggly lines, but they operate more like Swiss Army knives—multipurpose tools with complex structures and hidden features deployed only when needed. Like the proteins they help construct, these polymers fold into dynamically shifting 3D structures whose forms dictate their widely varying functions, including catalyzing chemical reactions and regulating gene expression.
But unlike proteins, the structural components of RNA molecules are little understood. Without deeper knowledge of these forms, we lack the ability to exploit these structures therapeutically.
“Because RNA is involved in essentially every aspect of gene expression, it’s also involved in every aspect of disease,” says structural biologist Steve Bonilla, who is at the forefront of efforts to identify its structures and the microscopic forces that mold them into shape. “If you can design small molecules or drugs that bind to a specific RNA 3D structure and somehow alter its shape or function, then it becomes a potential drug target for whatever process that particular RNA is involved in.”
“Because RNA is involved in essentially every aspect of gene expression, it’s also involved in every aspect of disease.”
For Bonilla, that could translate into new ways of combating infectious disease. His lab studies how 3D structures encoded in the genomes of positive-stranded RNA viruses such as dengue, Zika, and West Nile play a role in the recognition—and hijacking—of the host cell’s biochemical machinery. Blocking viral RNA from adopting the shape needed to commandeer the machinery might lead to new antivirals.
But it’s a long and winding road between our current understanding and the ability to develop those drugs. Part of what makes pinning down RNA structures such a challenge is that they are constantly changing. “RNA does not behave like a static object. Instead, it behaves like an ensemble of objects moving in concert with one another,” he says.
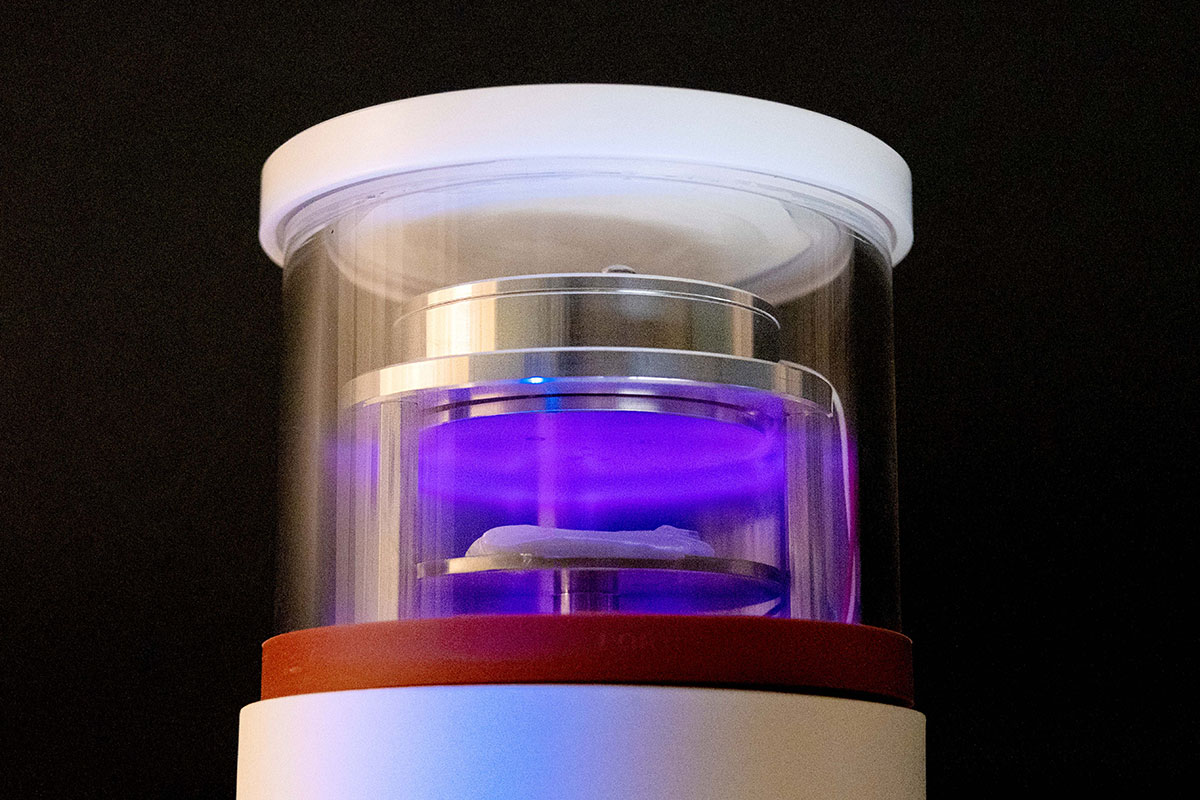
Bonilla, who heads the Laboratory of RNA Structural Biology and Biophysics, was one of the earliest researchers to use single-particle cryo-EM to capture the molecular choreography of small RNA 3D structures in viral genomes. Currently, he’s freeze-framing additional dynamic ensembles critical to their replication. He hopes stringing together these snapshots could eventually help devise strategies for disrupting viral life cycles.
That’s crucial, because while there are already thousands of RNA structures that need to be visualized, Bonilla believes many more await discovery. “There are many noncoding RNAs that we know exist, but we just haven’t figured out what their functions are,” he notes. “Once we know what form they take, we can begin to identify functions that are yet unknown.” –JP
Lamia Wahba
We know that genetic information is encoded in DNA and passed down to offspring. Changes, both adaptive and random, appear over time, morphing the species.
But scientists know DNA doesn’t explain everything. Lamia Wahba is one of them. As head of the Laboratory of Non-Canonical Modes of Inheritance, she studies alternate mechanisms for passing down biological information. RNAs of all kinds are increasingly being seen here as key players.
Her early research homed in on a small class of non-coding RNA molecules called piRNAs. “They have a very well-established role in maintaining genome stability by tamping down elements that might otherwise repeat uncontrollably, leading to disease,” she says. Through this work she discovered a new mode of nongenetic inheritance in roundworms, in which small RNAs that can silence specific genes are passed down.
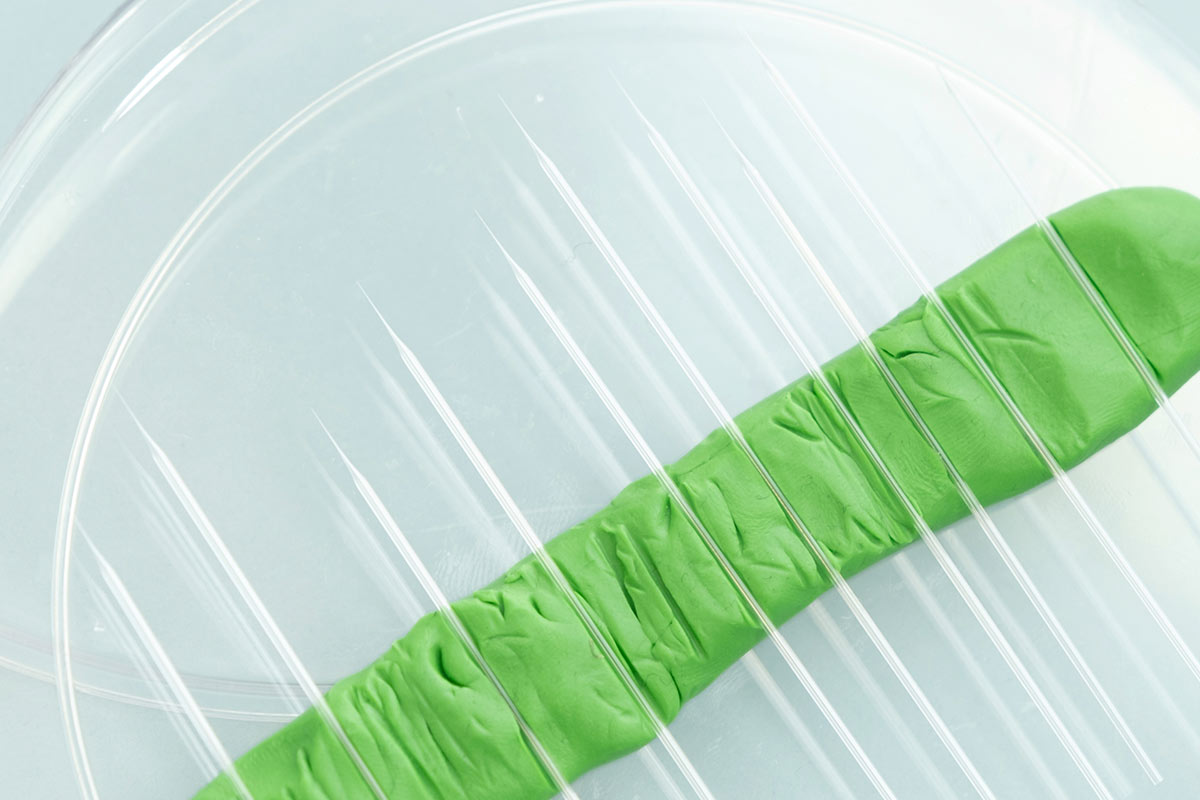
But much remains unknown about these functions of small RNAs. How is the process regulated across generations? What promotes it, what limits it, and what are the long-term implications?
These mechanisms can put evolution on a fast track, with dramatic changes appearing in a short period of time and immediately handed down to descendants, Wahba says. “This can crop up in microbial drug resistance and mutation-driven diseases, so understanding more about these mechanisms could help us find ways to counter their effects,” she notes.
Wahba’s research could help explain why so few genetic diseases have a definitive origin. “There are some diseases where the relationship is really one-to-one: If you have this mutation, you get this disease and can potentially pass it down to your children,” she says. “But for most, the relationship is much weaker, with a known link identified only about 20 percent of the time.” As for that missing 80 percent, Wahba suspects some of the culprits to be proteins, epigenetic chromatin marks, and foremostly RNAs.
“We may get to a place where we sequence not only someone’s genome but also their various epigenomes.”
Her findings may also improve CRISPR, the most promising gene-editing tool discovered to date. She’s identified a gene essential to the formation of three-stranded tangles called RNA:DNA hybrids, whose mixed nature makes them useful guides. “Boosting this gene’s output might increase CRISPR’s efficiency by helping the tool bind better to the target site,” she says.
Expanding our knowledge of these profound nuances of inheritance will lead us toward more precision in medicine, she believes. “We may get to a place where we sequence not only someone’s genome but also their various epigenomes, and that information collectively will give a much better predictability of their likelihood for certain diseases, and how we might be able to intervene,” Wahba says. “To me, that’s the next wave.” –JP