Feature
Even small brains make big decisions
Understanding choice is the next frontier in neuroscience
By Caitlin Shure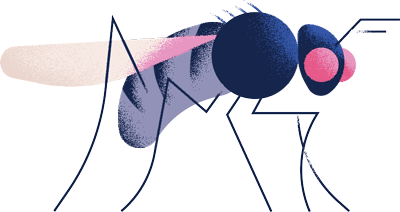
A tiny fruit fly ambles through a barren landscape. As the minutes go by and the temperature rises, she grows anxious to find food and shade. Then, along the horizon, she spots the sun—or something like it. The fly makes a decision: She will walk away from the glaring light and hope for the best.
Although she is not aware of it, the fly’s every step is being observed and recorded by a coterie of curious humans and a bank of sophisticated tracking equipment. The “sun,” it turns out, is a vertical bar illuminated on an LED display, part of a virtual environment. The ground is a pea-sized foam ball, floating on a cushion of compressed air, that spins as the fly traverses its surface. And this entire scene—the heat, the hunger, the bright light—has been carefully orchestrated by neuroscientists in the lab of Gaby Maimon to shed light on one of the trickiest questions in biology: how behavioral decisions are made.
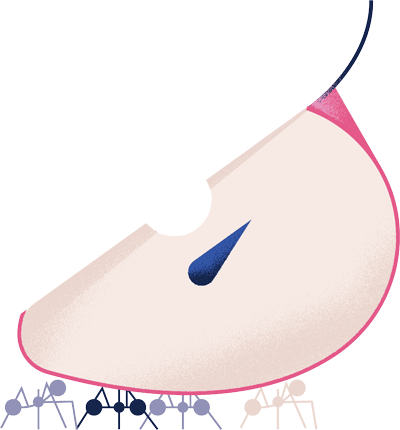
To be sure, scientists are still far from understanding how a nervous system makes choices—or, really, how it does anything at all—even on the relatively small scale of a fly brain. But increasingly, innovations in technology are making it possible to dissect brain computations at the level of molecules, cells, and neural networks, especially when applied to microcosmic systems such as fruit flies, worms, or ants.
“By working in a smaller brain with fewer neurons, you can more readily understand how cells guide behavior,” Maimon says.

In this respect, a parade of tiny critters is showing the way as neuroscience moves toward its next frontier. The simplicity of microscopic organisms allows scientists to make headway in understanding otherwise intractable aspects of cognition, knowledge that will one day be applicable to the study of all brains, including humongous ones like our own.
In that sense, our smallest peers in the animal kingdom might have grand things to teach us—including, perhaps, some of the basic rules that govern our thoughts and actions.
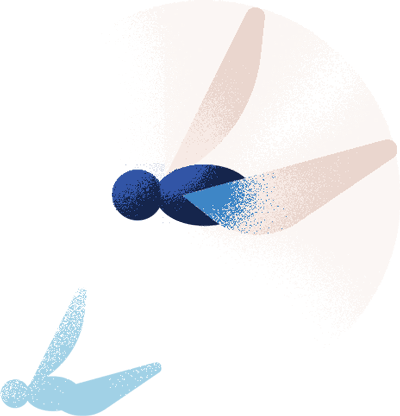
For animals, human and otherwise, life consists of a series of decisions—junctures that call for an individual to either fight or flee, hunt or hide, flirt or forget about it. Resolving such dilemmas requires the ability to detect what’s going on in the environment, process that information, compare the situation to past experiences, and behave in a way that is appropriate to the circumstances.
Which is to say, it requires a brain.
Brains can consist of millions of cells—or billions, at the high end of the spectrum. To navigate decisions, each cell must engage in its own kind of deliberation. Neurons repeatedly grapple with the same binary choice: either produce a burst of electrical activity, or don’t. In neuro lingo: fire or don’t fire.
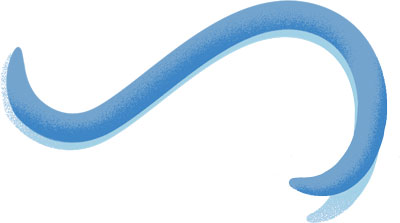
In theory, much of what you say or do can be boiled down to a network of firing brain cells. In practice, however, we don’t have a good picture of what such networks look like or how information flows through them. In other words, it is quite difficult to establish reliable relationships between what neurons do and what organisms do.
Further complicating matters, a given behavior can have more than one underlying cause, each involving the activation of a unique brain network.
In theory, much of what you say or do can be boiled down to a network of firing brain cells. In practice, however, we don’t know what such networks look like.
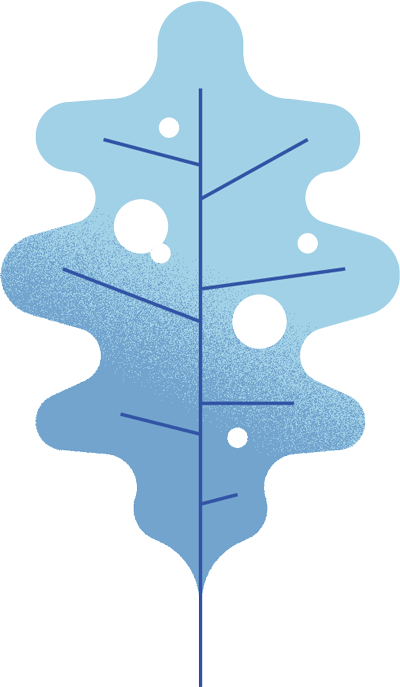
“If I decide to walk out the door, it may be because it’s hot in here. Or because I see my grad student outside the door. Or because I want to go get lunch,” says Maimon, who is head of the Laboratory of Integrative Brain Function. “Human brains and human motivations are complex. It’s an enormous task to tease apart that sort of complexity. So maybe you reduce the system a little.”
This is the idea behind the virtual environments Maimon’s lab has engineered. While striding on her spherical treadmill, the fruit fly is made to respond to a few carefully controlled cues—like temperature or light—that influence her navigational decisions. All the while, her brain is under a microscope that measures bursts of firing neurons.

The fruit fly is prepared to walk for meters if it means finding respite from the heat, and she has a plan: As long as she keeps the sun at a constant angle, she knows she’s walking in a straight line—a line that, she hopes, will eventually lead her to a more hospitable climate.
But when the LED “sun” confoundingly moves, the fly comes to believe that she has strayed from her intended path. She corrects, turning her body so that the sun is, once again, where she prefers it. Like other fruit flies before her, the animal has just consulted an internal signpost to make a navigational decision. And Maimon, along with Jonathan Green and Vikram Vijayan, two scientists in his group, has come one step closer to figuring out how the fly brain accomplishes as much.
Previous studies have shown that this fly species, Drosophila melanogaster, has a doughnut-shaped brain structure containing a set of neurons called E-PGs; as a fly turns its body, different E-PGs around the doughnut become active, indicating the fly’s orientation. The activity of E-PGs functions as a sort of compass needle for the fly.
Like the activity of most neurons, E-PGs depend on input from other cells. Just as a person might solicit advice from close confidants when facing a decision, deliberating neurons weigh input from their fellow neurons, communicating with one another through the release of chemicals known as neurotransmitters. Some of these chemicals are excitatory, meaning they nudge a neuron toward activation. Others are inhibitory, tilting the scale toward inaction. When receiving this oft-contradictory counsel, a neuron sums up the totality of its input to “decide” what to do next. If excitation exceeds inhibition, for example, it fires.
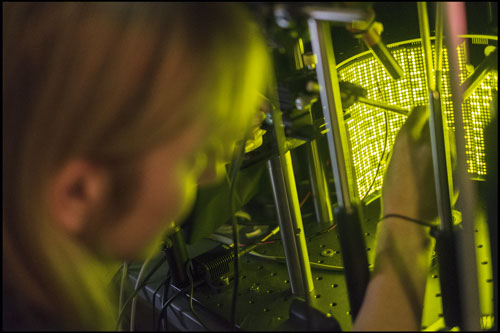
In 2017, Maimon’s lab discovered that a group of neurons, called P-ENs, monitor how fast a fly’s body is rotating and feed this information to E-PGs, influencing their decision to fire. In one experiment, the researchers stimulated brain cells that rotate the E-PG compass as a fly attempts to walk in a straight line. The insect, believing that she has deviated from her path, turns to realign herself.
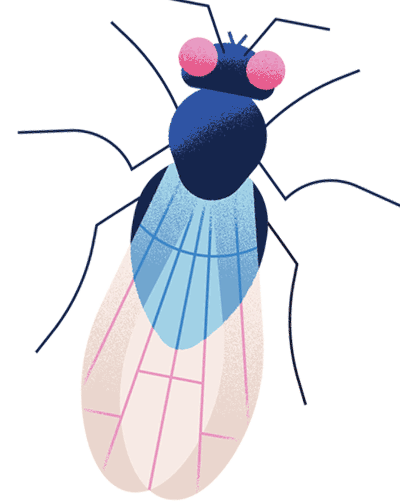
These and other of Maimon’s experiments illustrate how groups of neurons, like E-PGs and P-ENs, interact to generate an internal sense of orientation—a sense that influences which direction a fly turns, how hard it turns, and how quickly it walks forward. Maimon has also found that flies participating in this kind of experiment always reorient themselves in the most efficient way possible. “If you rotate the compass 30 degrees clockwise, then the fly rotates its body 30 degrees counterclockwise—it rarely takes the long route,” he says.
For a fly, the ability to walk in a straight line can mean the difference between starvation and a lovely lunch, between life and death. For Maimon and his colleagues, working with this organism provides a rare opportunity to pinpoint the precise neuronal processes that keep track of the animal’s goals and guide its behavioral decisions. Using the framework of navigation, his lab hopes to elucidate how chemical and electrical processes interact to give rise to basic aspects of memory and cognition—a big question that, he says, is most readily answerable in small brains.
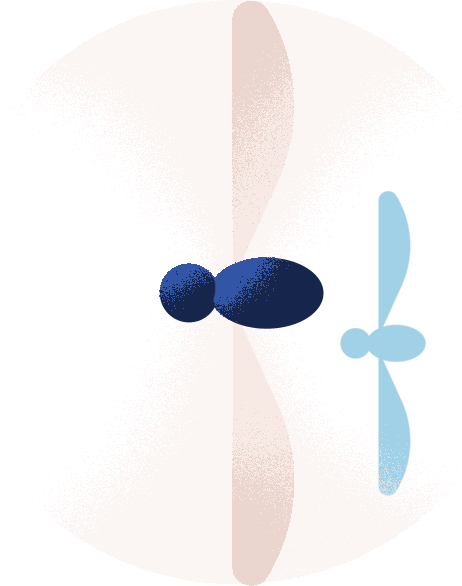
A few stories down, another fly navigates an arguably more difficult scene—the dating scene. Typically, male fruit flies have strict courtship criteria. They mate only within their own species, and they strongly prefer a female that hasn’t mated before. More than a matter of taste, this pickiness is a vital trait: A male’s choice of mate will affect the fitness of his offspring and, ultimately, the future of the species.
Under normal circumstances, a D. melanogaster would never be interested in, say, a D. simulans, even though females of the two species look indistinguishable and run in similar circles. Today, however, one particular fly finds himself attempting to mate with a ladybug. Objectively, this makes no sense. Among other problems, the ladybug is many times the fly’s size. It doesn’t go well.
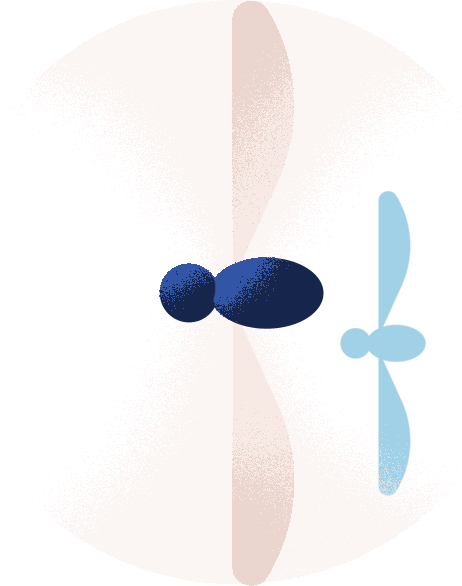
The fly, a resident of the Laboratory of Neurophysiology and Behavior, is under the spell of a high-tech love potion of sorts. Vanessa Ruta, head of the lab, has genetically engineered one of its neurons, known as P1, to fire when exposed to light, a technique called optogenetics. Using this approach, Ruta shows that every time the light gets turned on, so does the fly. P1 neurons control a fly’s decision to court, and they are capable of overriding the fly’s best judgment.
In nature, of course, P1 does not receive optical stimulation and D. melanogaster flies don’t mate with ladybugs. In fact, Ruta has found that P1 neurons become active only when a fly is in the presence of a female of the same species, suggesting that the neurons discriminate between appropriate and inappropriate objects of affection. Which, to Ruta, raises the crucial question of how P1 neurons carry out the discernment. How, she wondered, do approximately 20 male-specific cells evaluate the suitability of a potential paramour? And how do these processes differ across species?
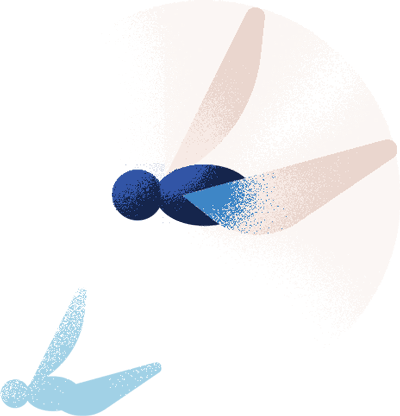
To find out, her team used the gene-editing technology CRISPR-Cas9, along with advanced imaging techniques, to compare the nervous systems of D. melanogaster and D. simulans. Specifically, they evaluated how the two species respond to the pheromones of melanogaster females. Here, the relative compactness of the fly’s brain was a crucial asset.
“Because the circuitry is concise and simple, we were able to trace activity cell by cell, and study neural architecture and function at every level,” says Ruta, the Gabrielle H. Reem and Herbert J. Kayden Associate Professor. “That allowed us to make explicit comparisons between the two species and pinpoint any variation.”
Through this exhaustive exploration, the scientists found that the sensory organs of the species were identical, as were their P1 neurons. However, the cells sending excitatory and inhibitory inputs to P1 neurons behaved differently. When researchers presented a D. melanogaster male with the pheromones of a D. melanogaster female, P1 neurons received predominantly excitatory input—urging the fly to make his move. But when they presented a D. simulans male with D. melanogaster pheromones, P1 neurons were strongly inhibited, splashing cold water on the entire interaction.
This outcome, says Ruta, makes good evolutionary sense, because if a D. simulans were to mate with a D. melanogaster, the couple would yield infertile offspring. The research contributes to her lab’s broader mission of examining the interaction between behaviors that are selected over the course of evolution, and those that are learned through individual experience—a “nature versus nurture” interplay that characterizes species of all sizes.
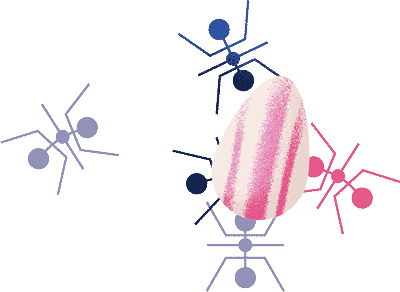
“You would think that it’s under your control to make the right decision,” she says. “But it turns out some decisions are so essential for the perpetuation of the species that they’re hardwired into the nervous system.”
When encountering an eligible female, a male fruit fly does not agonize over whether the insect in front of him is his soul mate, or even whether she shares his taste in overripe bananas. Rather, any deliberation about romantic compatibility occurs on a cellular scale: Upon detection of the female’s pheromones, the male’s P1 neurons receive excitatory input, they fire, and he pursues her. End of story.
That doesn’t mean, however, that small organisms amount to automatons. Nor does it mean that their decisions can always be explained by the presence of some specific stimulus in the environment. In addition to external cues, decisions can be driven by internal motivations.
“When we talk about decision making, we often talk about it as though the whole problem is the sensory information that’s in front of you,” says Cori Bargmann, the Torsten N. Wiesel Professor and head of the Lulu and Anthony Wang Laboratory of Neural Circuits and Behavior. “But a lot of the decisions that an animal, or a person, makes are driven by internal needs.”
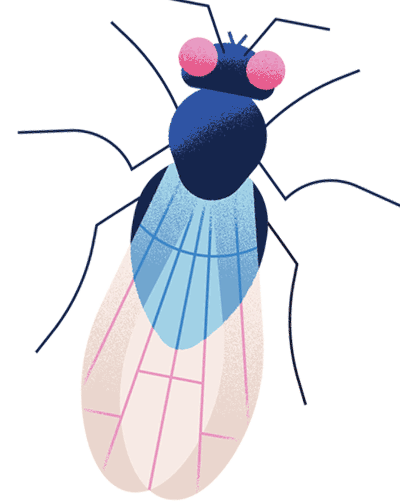
Consider Maimon’s meandering fly. Sure, its directional decisions were influenced by a fake sun and some brain stimulation. But the fly was also hungry—which is why it was so motivated to scurry along in the first place.
Hunger is a powerful behavioral motivator for all animals, including the microscopic roundworms that Bargmann studies. Her lab houses a collection of petri dishes filled with these organisms, known as Caenorhabditis elegans, whose nervous system consists of a mere 302 neurons, all of which have been thoroughly mapped. Exploiting the simplicity of this system, Bargmann and her colleagues have been able to investigate highly specific relationships between genes, brain activity, and behavior.
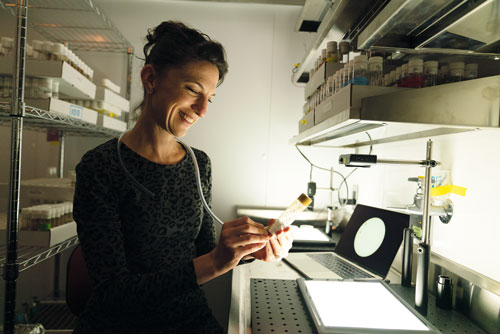
C. elegans spend most of their time investigating where to find something to eat. They perpetually scan their surroundings for edible bacteria; and if they fail to find any, they go to new lengths—quite literally—to satisfy their cravings. At the first hint of hunger, a typical worm surveys the region where it last found sustenance, a strategy called local search. This can go on for up to 20 minutes, as the worm checks and rechecks its proverbial cupboard. Eventually, however, the animal abandons this tactic and goes hunting in foreign terrain—raising the question of what changed in its brain to incite this new behavior.
Because the worms continue to search locally long after food is removed from their surroundings, Bargmann suspected that they’re dwelling upon some kind of memory—nostalgia for meals past that compels them to search in a spot previously brimming with bacteria. The decision to switch hunting strategies, then, could be explained by the eventual waning of that memory.
“You would think decisions are under your control. But some are so essential for the perpetuation of the species that they’re hardwired into the brain.”
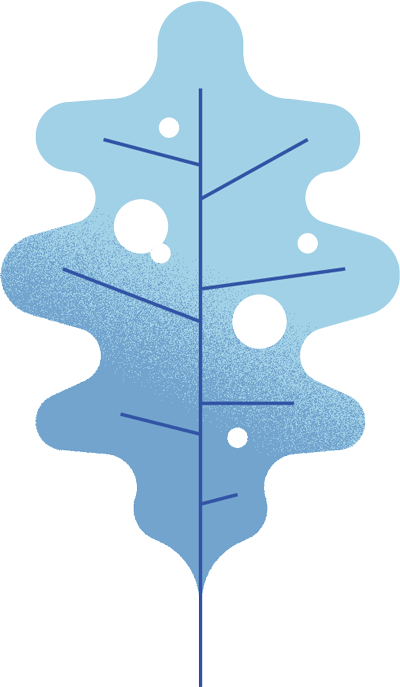
Hoping to determine how the worm brain might store this kind of information, Alejandro López-Cruz, a member of Bargmann’s lab, identified a receptor, known as MGL-1, that is involved in detecting food. Activation of MGL-1, the researchers found, leads to a circuit change that modifies the behavior of neurons for minutes at a time—sustaining food-related memories, and keeping worms close to home. When this chemical memory eventually fades, the animals move on from their comfort zone and forage elsewhere.
Though it is perhaps intuitive that memories should affect an animal’s decisions, how this process works on a molecular scale is hardly straightforward. But with increasingly sophisticated tools to exploit the C. elegans nervous system, Bargmann’s lab has a unique opportunity to identify the biological machinery through which past experiences guide present decisions. Building on this work, the researchers hope to better understand how memories form and degrade, and how those memories affect behavior.
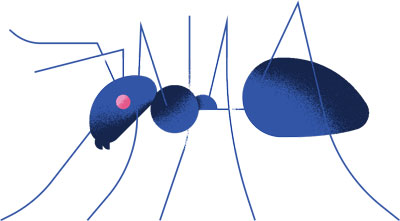
To make an informed decision, a brain must be attuned to the goings-on of the environment, its own needs, and its memories. And in case that isn’t enough to deal with, there’s also the matter of other individuals.
For ants, who are often literally walking all over each other, it is especially important that everyone stays in step. Luckily, ants are pretty sensitive to the needs—or at least the pheromones—of their peers.
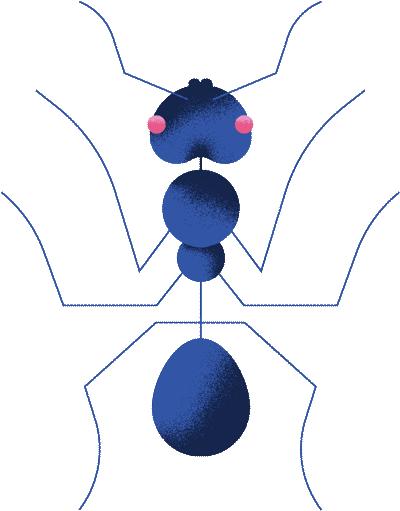
The clonal raider ant Ooceraea biroi is a particularly social species: Members of a colony collectively find and retrieve food and take care of their offspring. Daniel Kronauer, head of the Laboratory of Social Evolution and Behavior, has developed elaborate techniques for studying these miniature collaborations. Using an automated tracking apparatus and a vibrant color-coding system, he can monitor more than 100 different colonies at a time. Employing this approach, his lab has demonstrated, among other things, the importance of social cues in O. biroi behavior.
Kronauer has observed, for example, that when larvae are around, ants stop laying eggs and instead tend to the kids that they already have. Further investigating this behavior, his team learned that the presence of larvae reduces expression of a gene coding for insulin—a signaling substance that, in ants, promotes reproduction. In other words, a social cue, the presence of ant larvae, alters levels of a neurochemical, insulin, which controls a decision, to halt reproduction. In establishing this kind of link between sociality and biology, says Kronauer, the advantages of a small nervous system are myriad.

“Because ant brains are relatively simple, it is easier to see how a molecule like insulin is affecting the animal, and what aspects of behavior, exactly, are being modulated,” he says. “In ants you are also able to observe effects that might be too subtle to detect in bigger organisms.”
Of course, being a responsible member of the O. biroi community requires a lot more than occasional babysitting. Ants must coordinate the activity of not just a nuclear family, but an entire colony, which can consist of hundreds of individuals. Often, every ant in a colony makes the same decision simultaneously—requiring remarkably efficient signaling both among the cells within an individual brain, and between brains.
Asaf Gal, a postdoc in Kronauer’s lab, is studying the dynamics of this kind of groupwide behavior. Specifically, he is investigating how O. biroi respond to increases in temperature, and how this behavior changes as group size increases. He has found that when a solo ant is exposed to rising heat, it scurries away almost immediately. When in a group, however, that same ant will take longer to make a move—and it does so in synchrony with the rest of its colony. Gal has also found that as group size increases, so does the amount of time it takes for a colony to start relocating—but only as long as the temperature change is moderate. When faced with a more dramatic heat wave, he notes, large colonies disperse as quickly as small ones.
“You only see this social phenomenon in borderline cases—when the temperature change is not too extreme,” says Gal. “The group’s decision is slower in these circumstances because there are opposing forces: Some ants feel it’s time to go, but others disagree. This suggests that the ants are communicating with each other, probably through pheromones, to figure out what to do.”
When enduring a still-tolerable hot spell, an ant might release a pheromone suggesting that it’s starting to get a bit too warm for comfort. As the temperature continues to increase, more and more ants emit this pheromone, and their peers pick up on the signal. Eventually, accumulation of these signals reaches a threshold that prompts the entire colony to march to a cooler climate. It’s not unlike the process by which individual brain cells detect chemicals released by their neighbors before making a determination to fire (see “Decision making: How it works,” above).
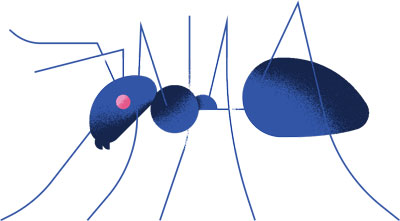
In this context, an ant is at once an individual and a node in a larger processing system—the colony. And within an ant itself is a smaller processing system, the brain, with neurons that perform calculations of their own. In this way, ant behavior illuminates multiple scales of biological deliberation—scales that confuse our assumptions about when, where, and by whom decisions are made.
In casual parlance, a decision connotes a conscious choice, sometimes accompanied by a fraught period of deliberation: Should I buy this house? Should I marry this person? Should I cut my hair?
These big questions, however, represent only a slim portion of the decisions that the brain faces. Because underlying everything that you do or think is a flurry of microdecisions—neuronal computations made in the absence of conscious consideration.
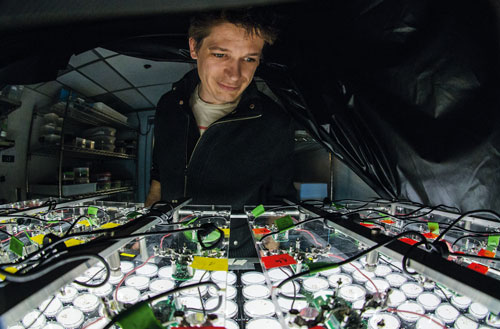
By analyzing the decisions of individual cells, small-organism researchers can define direct links between genes and neurotransmitters, neurotransmitters and firing patterns, firing patterns and behavior. As such, little animals are laying the groundwork necessary for neuroscientists to understand how all brains, big and small, function at the most rudimentary level.
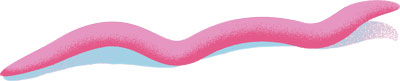
In the immediate, research on tiny critters will not resolve how we arrived at our choice of haircut, house, or spouse. But it provocatively calls into question whether we choose anything at all. Research on humans is complicated not just because our brains are so inconveniently big but because when we consider our own behavior, we often get distracted by abstract concepts like consciousness, love, and freedom. In the context of small organisms, it is easier—conceptually and experimentally—to see decisions for what they really are: the upshot of cells firing or not firing due to the influence of chemical input.
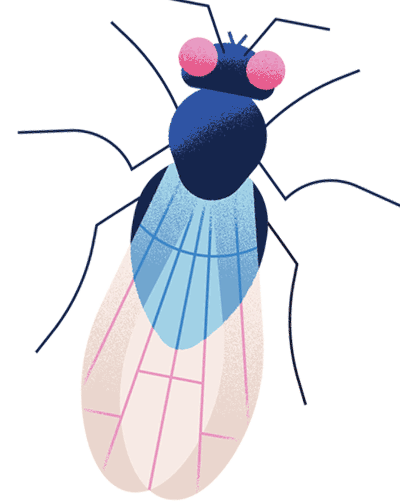
“People tend to think of ants as these programmed robots,” says Kronauer. “At the same time, we think about ourselves as these free-willed, free-spirited organisms. And that dichotomy is probably not correct.”
In a sense, the attribution of decisions to chemical-induced moods or hardwired circuitry may seem at odds with the kind of autonomous actors that we fashion ourselves to be. On the other hand, we may rest assured knowing that even our most regrettable choices adhere to some neuronal logic—complex cellular calculations that we are only just beginning to understand.