Feature
All the world’s genes, at our fingertips
A bit of genetic trickery, borrowed from bacteria, has made gene editing easy. The question now is how to make good use of CRISPR.
By Alexander GelfandStanding before a collection of plastic vials and petri dishes, Luciano Marraffini, head of the Laboratory of Bacteriology, surveys the tools that he uses to study bacterial evolution.
Some of the vials, Marraffini explains, contain plasmids: circular bits of DNA that travel from one bacterium to another, spreading useful genes as they go. (Bacteria don’t reproduce sexually, so they must rely on other means of refreshing their gene pools.) Other vials contain viruses known as bacteriophages—phages, for short—that kill bacteria, yet may also pass them genes that increase their virulence.
CRISPR is not the first technique to modify genes, but it’s by far the most flexible and useful.
As Marraffini talks, Philip Nussenzweig, a graduate student in the lab, pulls a petri dish from a refrigerator hidden beneath a countertop. The dish is loaded with Escherichia coli, the gut bacterium that causes traveler’s diarrhea and, on rare occasions, kidney failure; and also with Staphylococcus aureus, a microbe that produces deadly infections of the heart, blood, lungs, and bones.
Lurking inside them all is yet another tool. It is called CRISPR (pronounced “crisper”) and it is the most powerful means of manipulating genes that the world has ever seen.
Biologists have long been able to alter genes using a number of techniques, and those methods of genetic engineering have played a vital role not only in laboratory research, but also in applications such as the creation of genetically modified crops, and of plants and microorganisms capable of producing drugs and vaccines.
Around a decade ago, however, scientists discovered a new class of gene-editing tools that allowed them to make highly specific DNA changes much more accurately and efficiently than ever before. And CRISPR, which exists in nature and was adapted only recently to form the basis of a new gene-editing technology, has provided biologists with the most flexible and widely applicable gene editor thus far. Over the past several years, researchers have used it to tweak the DNA of dozens of organisms, from wheat and trees to cows and chimpanzees.
At Rockefeller alone, laboratories working on a vast range of human diseases keep finding new uses for the technology (see “Three ways CRISPR could advance medicine and help people,” right). Meanwhile, Marraffini, who played an important role in the development of CRISPR, continues to conduct experiments on bacteria and phages to elucidate the inner workings of the system.
“In a way, we’re playing with this thing,” he says. “And that’s what makes it fun.”
That may be. But it is a kind of play that has already revolutionized biological research. And the fun is only just beginning.
In the late 1980s, researchers at Osaka University in Japan identified curious repeated sequences in the DNA of E. coli—sequences that were interspersed with other, non-repetitive stretches of genetic material.
Those repeated sequences and their intervening spacers were dubbed CRISPR, for “clustered, regularly interspaced, short palindromic repeats.” Scientists soon found CRISPR in many different bacteria and in other single-celled organisms called archaea; and they eventually figured out that these CRISPR systems formed part of a bacterial immune system that fended off attacks from phages.
For example, investigators determined that the spacers represented snippets of DNA captured from phages, plasmids, and other foreign sources of genetic material. They surmised that those snippets functioned like genetic mug shots that allowed bacteria to recognize phages that had previously attacked them. And they gathered that somehow, the infected bacteria were able to render these returning phages harmless with the help of special CRISPR-associated (Cas) proteins (see “CRISPR: How it works,” below).
Nonetheless, they still did not know quite how these CRISPR-Cas systems—and there are many different types—accomplished all of that. Their best guess was that CRISPR-Cas neutralized invading phages by targeting their RNA, a close chemical relative of DNA that phages rely upon to replicate themselves. The idea initially seemed plausible, but in 2008, Marraffini published work demonstrating that the CRISPR-Cas system he’d identified in the bacterium Staphylococcus epidermidis was in fact targeting DNA.
This research—which Marraffini began in his spare time while completing his Ph.D., and which subsequently formed the basis of his postdoctoral work with molecular biologist Erik Sontheimer, at Northwestern University—represented a major breakthrough in understanding how CRISPR-Cas actually worked. And in a phrase that today seems remarkable both for its restraint and for its prescience, Marraffini and Sontheimer suggested that, if it could be put to use elsewhere, the ability of CRISPR-Cas to target DNA might have “considerable functional utility.”
Less than a decade later, the utility of CRISPR-Cas is no longer a matter of speculation. And Marraffini is regarded as one of the leading figures in a gene-editing revolution that is poised to transform fields ranging from medicine to industrial agriculture.
Marraffini’s 2008 prediction turned out to be prophetic, a self-fulfilling prophecy, really, since after coming to The Rockefeller University in 2011, he himself would help establish that a particular CRISPR-Cas system called CRISPR-Cas9—so named for the Cas9 protein, which cuts through double-stranded DNA as neatly as a molecular scalpel—could be modified in the lab to make precise edits in the genome of any organism.
In 2012, Marraffini and his team at Rockefeller, along with Feng Zhang at the Broad Institute, were among the first to demonstrate that this programmable form of CRISPR-Cas9 could be used to edit genomes in mice and human cells. They used it to alter the function of specific genes or put them out of action altogether by making multiple edits to their DNA sequences; and they inserted chunks of new genetic material into existing genomes.
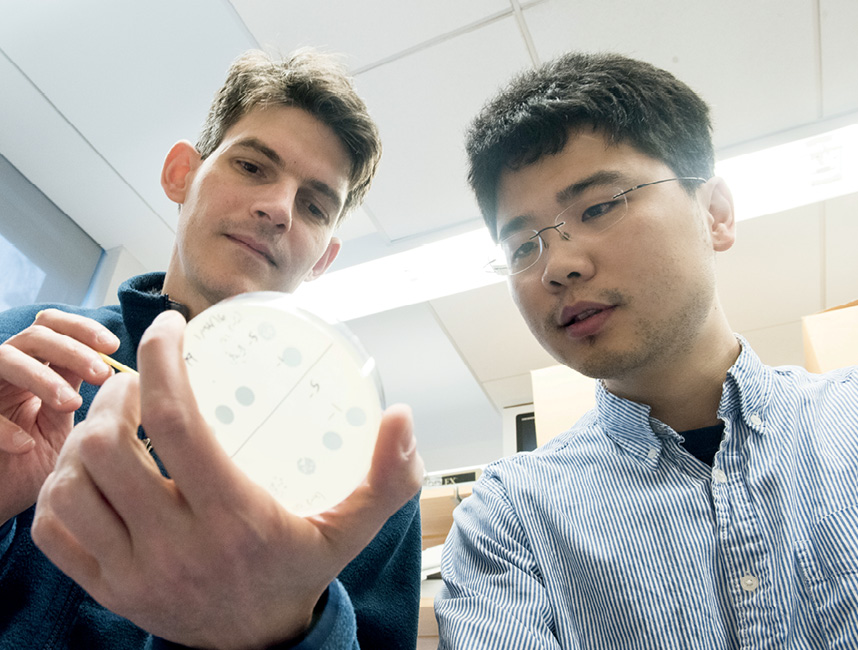
These experiments helped show that CRISPR-Cas9 could be further modified to repress or activate genes without actually cutting them—a potentially useful trick for studying organisms in which different genes are switched on and off at different times, and for designing synthetic ones that do the same.
In yet other experiments, Marraffini and his team at Rockefeller programmed CRISPR-Cas9 to target only virulent and antibiotic-resistant strains of S. aureus—the same bacteria that cause antibiotic-resistant infections in hospital patients—in lab mice, thereby demonstrating that the technology could be used to selectively kill bad bacteria while leaving good bacteria alone. They even used it to stop the bacterium Streptococcus pneumoniae, a potentially lethal microbe that can cause pneumonia, meningitis, and a host of other infections, from switching from its non-virulent form to its virulent one.
Scientists have since employed CRISPR-Cas9 to cure muscular dystrophy in mice; make human stem cells immune to HIV; and overcome at least some of the genetic challenges that have thus far prevented doctors from transplanting pig organs into people. CRISPR-Cas9 is not the first tool to make it possible to tinker with patients’ genes in order to cure diseases; other forms of gene therapy have been developed, and some are already being tested in clinical trials. But none of its competitors are as versatile or as easy to use as CRISPR-Cas9. As a result, just about everyone with an interest in manipulating DNA seems to have jumped on the CRISPR bandwagon.
“I have enough gray hair to look back 30 years, and this is just astonishing,” George Q. Daley, a stem cell biologist at Harvard Medical School, said of the field during a seminar about CRISPR that took place at Rockefeller last year.
When asked how long it might take to see CRISPR-driven therapies in the clinic—treatments that in the near term could be used for sickle cell anemia, and that someday might eradicate genetic diseases like cystic fibrosis or reduce the risk of complex ones like Alzheimer’s—Daley, who directs the Stem Cell Transplantation Program at Boston Children’s Hospital and is himself using the technology to seek better therapies for patients with bone marrow disease, did not hesitate.
“Maybe even within two years,” he responded. “But certainly within five.”
In fact, the rapid progress of scientists’ ability to use CRISPR-Cas9 to engineer living things is raising concerns that it may be outstripping society’s ability to determine whether doing so is safe or ethical.
The scientific community is itself divided over this issue. Some researchers, for example, are keen to use CRISPR-Cas9 to modify animals such as mosquitoes in order to combat public health threats like malaria and Zika. Others, however, worry that releasing such modified organisms into the wild could have unforeseen consequences for natural ecosystems.
And then there is the issue of determining how, and when, to use CRISPR-Cas9 in people.
In March 2015, for example, a group of prominent biologists called for a moratorium on using CRISPR-Cas9 to modify human eggs, sperm, and embryos. Such modifications, known as germline edits, could be passed down to future generations. And they raise the specter of what Daley calls “the dark side” of CRISPR-Cas9: the prospect of a new era of eugenics, of a brave new world divided between genetic haves and have-nots. Yet in April of that same year, Chinese researchers revealed that they had already used CRISPR-Cas9 on human embryos. (The embryos themselves were nonviable, and could not have gone on to become babies.)
In response, leading scientists in the field, under the auspices of a number of international scientific organizations, convened a global summit on human gene editing in Washington, D.C., in December 2015. In addition, the U.S. National Academy of Sciences and the National Academy of Medicine commissioned a comprehensive study by an international committee, of the legal, ethical, and social implications of the technology. The committee is preparing a formal report that will include policy recommendations on its use and regulation (see “The future opportunities—and conceivable dangers—of CRISPR,” below).
Yet despite these caveats, movement toward human applications proceeds apace: Last June, the Recombinant DNA Advisory Committee, which reviews all human gene therapy protocols in the United States, approved the first such protocol involving the use of CRISPR-Cas9. And the technology continues to generate widespread excitement over the promise it holds for both basic scientific research and for a broad range of applied fields including drug development, public health, and agriculture.
To marraffini, the story of CRISPR is “a testament to the value of basic biology for society.” He was drawn to the topic, which had no obvious applications for improving human health, out of sheer curiosity: a desire to understand how bacteria defend themselves, acquire genetic material, and evolve. Yet now, in what by scientific standards is the mere blink of an eye, the practical applications of CRISPR seem almost without limit, and Marraffini’s own work with bacteria exemplifies the dual promise of the technology: In addition to explaining how bacteria evolve, his research could one day lead to better antibiotics, or help impede the spread of antibiotic resistance.
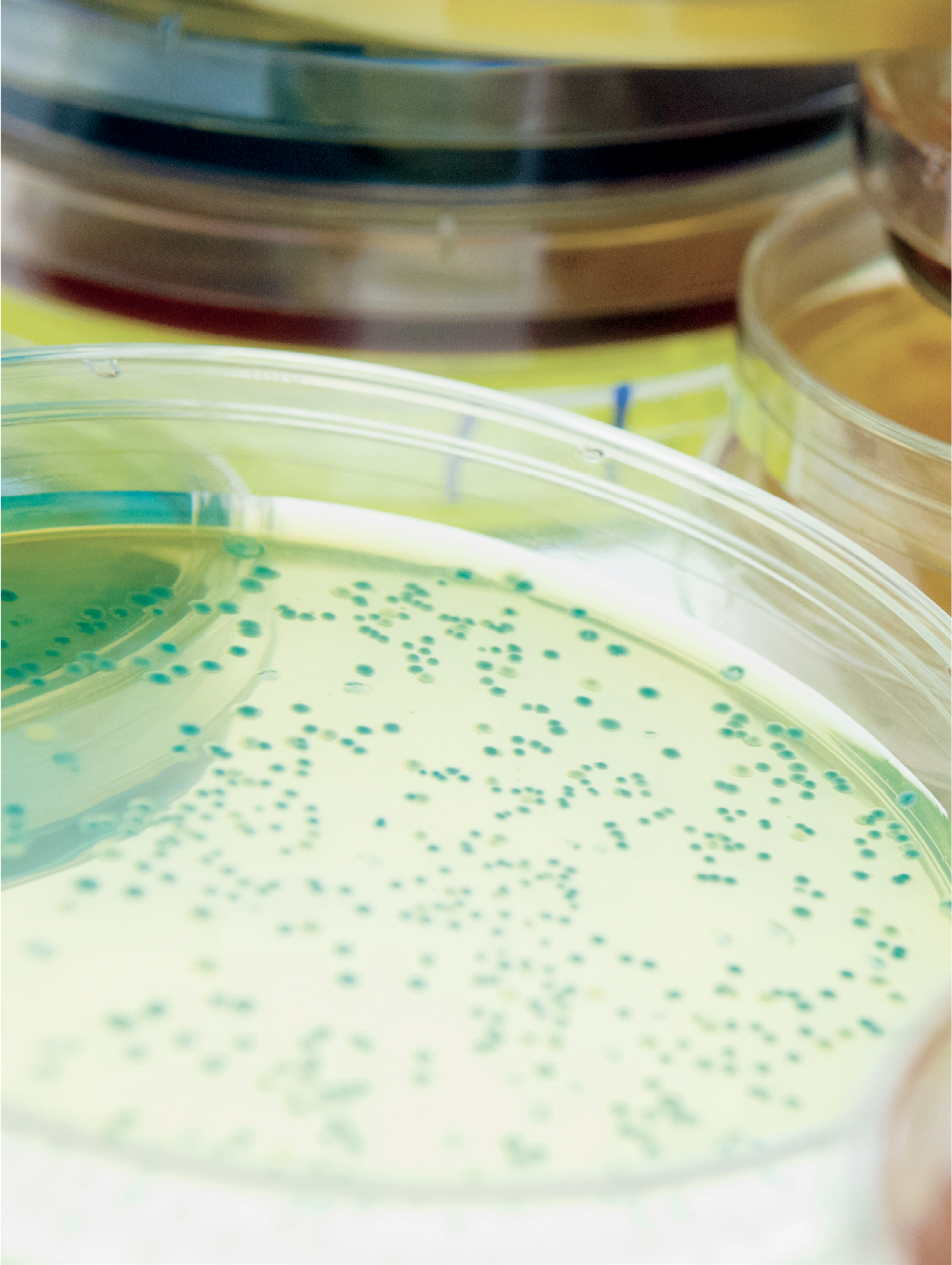
Much the same might be said of the CRISPR-driven work being done in Leslie B. Vosshall’s lab, where fundamental research on a major disease vector could eventually help control one of the world’s great scourges.
Vosshall, who is Robin Chemers Neustein Professor, and her team study Aedes aegypti, the mosquito that transmits the yellow fever, dengue, West Nile, and Zika viruses. Gram for gram, it is one of the most dangerous animals on the planet. And it finds its human prey, as well as the standing water in which it lays its eggs, by relying on a complex system of sensory cues that include humidity and heat, the chemicals in our sweat, and the carbon dioxide in our breath.
Historically, mosquitoes have been extremely difficult to modify genetically. Today, however, Vosshall and her team are using CRISPR-Cas9 to investigate how Ae. aegypti’s biology drives its behavior: how the insect is drawn to us by specific molecules in our body odor; how it detects the presence, and even the quality, of the water where it deposits its offspring; and why it is attracted by the lactic acid we excrete but repelled by a chemical such as DEET.
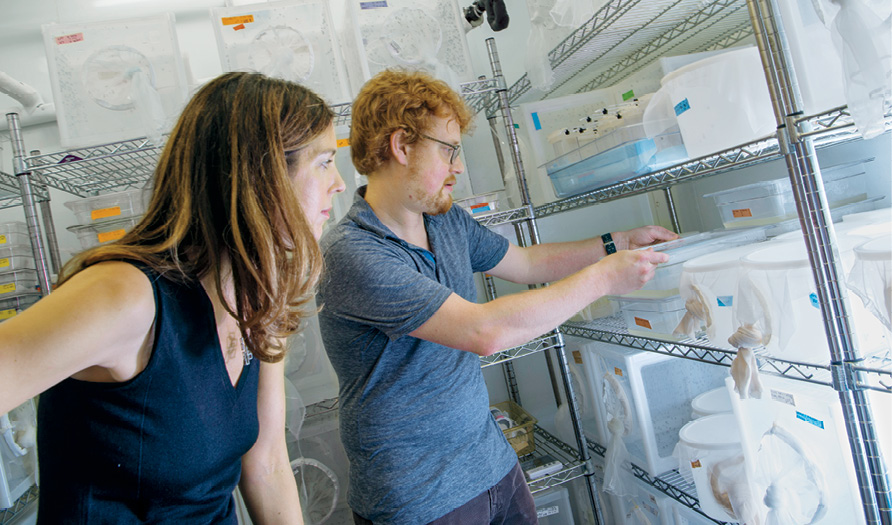
But in manipulating one mosquito gene at a time, they are not only unpacking the mysteries of an exquisite piece of biological machinery that has evolved over millions of years. They are also generating insights that could be used to make better mosquito repellents, or limit the number of these blood-sucking disease vectors. And they are developing broadly applicable methods that other researchers are already adapting to other insects, such as ants, which were previously inaccessible to genetic modification.
In the past, Vosshall and her team relied on other gene-editing technologies with obscure names (TALENs, zinc-finger nucleases) to create mutant mosquitoes that lacked particular genes, such as the ones that regulate the ability to detect carbon dioxide or certain kinds of odors. They then ran experiments on the insects in order to determine what role those missing genes played in guiding Ae. aegypti to us. Would odor-blind mutants still find and bite people, for example? Or would they be rendered harmless? (The answers to those questions turned out to be “yes” and “no,” respectively.)
But where those earlier methods were tricky, slow, and expensive, CRISPR-Cas9 is straightforward, fast, and economical. Ben Matthews, a postdoctoral fellow who leads the CRISPR-Cas9 program in the Vosshall lab, recalls attending a genome-engineering conference in 2013, soon after Marraffini and others began publishing the first papers describing the many practical applications of CRISPR-Cas9. Even at that early date, Matthews’s colleagues told him that the technology was “too easy not to try.”
They were right. Obtaining the materials from a commercial lab to make a mutant mosquito using earlier techniques cost between $5,000 and $25,000 and took three months. Today, preparing the equivalent materials in-house using CRISPR-Cas9 takes one week and costs roughly $25. “It’s ridiculously cheap,” Vosshall says.
The insectary where Vosshall and her team rear their research subjects—a warm, humid room packed floor-to-ceiling with water-filled trays containing larval Ae. aegypti and mesh boxes containing adults—is now chockablock with mutant mosquitoes that have had various genes knocked out or edited in more complex ways. Being able to operate on that kind of scale, and to make so many different kinds of mutants so easily and so quickly, says Matthews, “opens the doors to experiments that we couldn’t even really have dreamt of 10 years ago.”
One of Vosshall’s goals, for example, is to insert a gene into Ae. aegypti that would cause the mosquito’s neurons to glow when stimulated by sensory input—a complicated bit of genome editing that would bring her lab closer than ever to cracking the code of how the insect’s intricate neurosensory system responds to odors, humidity, and other cues.
“We’ve made halting attempts in the last five years,” she says. “But it looks like there will soon be a breakthrough.”
Two floors below Vosshall’s mosquito reservoir, Marraffini continues to play with his own, considerably smaller mutants: modified versions of the plasmids, phages, and bacteria that led to the CRISPR revolution in the first place. And discoveries continue to accrue: Just recently, he and his team identified a CRISPR-Cas system that targets not only DNA, but RNA, as well—an ability that would appear to confer an evolutionary advantage upon the bacteria that enjoy it.
At the moment, Marraffini sees no obvious practical application for this switch-hitting form of CRISPR-Cas. But then again, the researchers who first discovered CRISPR nestled in the DNA of E. coli nearly 30 years ago had little inkling of its considerable functional utility. And as Marraffini points out, if there’s one thing that working with CRISPR has taught him, it’s never say never.